Biologie.univ-mrs.fr
Annu. Rev. Genet. 2002. 36:153–73
° 2002 by Annual Reviews. All rights reserved
STUDYING GENE FUNCTION IN EUKARYOTES BY
CONDITIONAL GENE INACTIVATION
Manfred Gossen1 and Hermann Bujard2
1Max Delbr¨uck Centrum, Robert-R¨ossle-Strasse 10, D-13125 Berlin, Germany;e-mail: [email protected]; 2ZMBH, Universit¨at Heidelberg, Im NeuenheimerFeld 282, D-69120 Heidelberg, Germany; e-mail: [email protected]
Key Words tetracycline-controlled expression system, site-specific recombinases,
transgenic animals, conditional overexpression, knock-in mice
■
Abstract The prospect of specifically controlling gene activities in vivo has be-
come a defining hallmark of many model organisms of biological research. Where once
the aim was to gain control over gene activities using endogenous control elements,
new technologies have emerged that owe their remarkable specificity to heterologous
components derived from evolutionarily distant species. This review highlights in-
ducible transcriptional systems and site-specific recombination. Their quantitative and
qualitative characteristics are discussed, with examples of how recent developments
have expanded the spectrum of cells and organisms that are now accessible to genetic
dissection of unprecedented precision. Transgenesis has already converted the mouse
into a prime model for mammalian genetics. Combined with the new approaches of
conditional activation or inactivation of genes, this model has opened up new horizons
for the analysis of gene function in mammals.
Site-Specific Recombination . . . . . . . . . . . . . . . . . . . . . . 155Tools for the Transcriptional Control of Gene Activity . . . . . . . . . . . 155Controlling Transcription via Tetracyclines . . . . . . . . . . . . . . . . 157
CONDITIONAL GENE ACTIVATION IN
S. CEREVISIAE
TARGETED CONDITIONAL MUTANTS OF
CONDITIONAL GENE ACTIVATION IN THE MOUSE . . . . . . . . . . . 162
Controlling Dominant Negative Gene Products . . . . . . . . . . . . . . 163Surviving Development by Conditional Transgene Expression . . . . . . . . 164Inducible Knock-ins . . . . . . . . . . . . . . . . . . . . . . . . . 164Inducible Knockouts . . . . . . . . . . . . . . . . . . . . . . . . . 165
Experimental approaches that have been developed and refined over the past decadenow allow us to conditionally modulate individual gene activities in eukaryotes ina highly specific, temporally defined, and cell-type restricted manner. Analysis ofthe accompanying phenotypic changes has provided new insights into numerousbiological mechanisms and processes hitherto not amenable to genetic dissection.
There have been two basic strategies: The first targets genes directly by site-specific recombination, resulting in activation, inactivation, or alteration of thegene of interest; the second aims at quantitatively and reversibly controlling agene's function, generally leaving the endogenous gene itself untouched within itsgenetic context. In this chapter, we emphasize concepts, with specific examples toillustrate methodological progress in the field, and summarize recent reports thatoften provide impressive new insights.
The most important single precondition for the sensible application of gene
inactivation strategies is specificity: Interference should be strictly limited to thegene under study. This demanding goal has been reached, to differing extents,by use of heterologous components or by specific alterations of endogenous pro-teins. Systems derived from prokaryotes, evolutionarily the most distant, meet thiscriterion and are therefore the most broadly applied. The Cre/Lox recombinationsystem of phage P1 of
Escherichia coli has been used very successfully in eu-karyotes, and the conditional knockout approach in transgenic mice has yieldeda wealth of information. In the second strategy, elements from prokaryotes seemto provide the highest specificity when utilized for controlling gene expression inthe eukaryotic cell, as exemplified in the transcription control system based onelements of the tetracycline (Tc) resistance operon (
tet operon) of
E. coli. Whenthe two basic strategies for modulating gene activities—recombination and inter-ference with gene expression—are compared, genetic analysis via temporally andspatially restricted alteration of a gene by site-specific recombination has provento be very powerful. Nevertheless, the potential of reversibly interfering with agene's activity, for example at the level of transcription, adds another level of so-phistication to the study of gene function in vivo. As is shown below, the ability toreversibly perturb a system permits not only comparison between the normal andthe perturbed state, but also analysis of the system's reactions after interferencehas ceased and during repeated cycles of gene inactivation/reactivation.
In the discussion that follows, we briefly point out the principles underlying
different approaches, generally referring to other reviews covering the various areasof research. We touch only briefly on the straight recombination approach via theCre/Lox and the yeast-derived Flp/FRT system because this topic has already beencovered by several excellent recent reviews (36, 39, 56). In our view, an essentialfeature of a "truly conditional" interference with a gene's activity is reversibilityof the induced perturbation. Hence we focus on controlled gene expression anddiscuss, in addition, the emerging synergies between this approach and site-specificrecombination.
CONDITIONAL GENE INACTIVATION
Despite the wealth of valuable information gained by controlling gene activ-
ities in cultured cells of various origins, we restrict ourselves to applications inmodel systems where genomic loci can be targeted in a defined manner and which,therefore, hold particularly great promise for the study of gene function in vivo:
Saccharomyces cerevisiae, the chicken B cell line DT40, and the mouse.
At present, the site-specific recombinases Cre (58) and Flp (51) are most frequentlyused for modulating gene activities via recombination. They target their specific 34-bp recognition site, loxP and FRT, respectively, and catalyze a recombination eventwithin these sites (Figure 1). As no cofactors are required for this recombination,both systems function in a heterologous environment, and after the temperature op-timum of Flp has been adjusted to 37◦C (8), both recombinases function efficientlyin mice, for example. Both systems allow DNA segments to be inverted or deleted in
cis or to be connected in
trans. Thus, besides conditional gene (in)activation, thereare several other applications such as chromosome engineering (84), recycling ofselectable markers (1), etc. Here, we sketch in brief the most generally applied ap-proach whereby genes are activated or silenced by deleting DNA fragments locatedbetween directly repeated recombination sites. In most cases, loxP/FRT sites areplaced in introns. Therefore, the respective gene will generally exhibit wild-typeproperties until the gene or gene parts are deleted. Thus, when a mouse containinga "floxed" or a "flrted" gene is crossed with an animal expressing the respectiverecombinase under the control of a tissue specific promoter, recombination willoccur only when the promoter driving the
cre or
flp gene becomes active withinthe developmental program of the mouse. Obviously, in many cases this approachallows potential embryonic lethality to be circumvented and leads to a temporallyand spatially well-defined genetic alteration. Both recombination systems show anexquisite specificity that has been documented in a large number of experiments.
Nevertheless, an increasing number of reports have described degenerate loxPsites in mammalian genomes that can be targeted by the recombinases in vivo andin vitro, albeit with low efficiency (40, 59, 64, 70), leading to undesirable effects.
Therefore, high constitutive levels of Cre or Flp should be avoided. Controlling theactivity of the recombinases, as is discussed below, is an alternative that expandsthe applicability of the recombination principle, even though it is more demandingexperimentally.
Tools for the Transcriptional Control of Gene Activity
The conditional transcription systems briefly outlined below either repress or ac-tivate promoters via "synthetic," i.e., specifically designed or heterologous, trans-cription factors. The first repression system modeled according to the prokaryotic
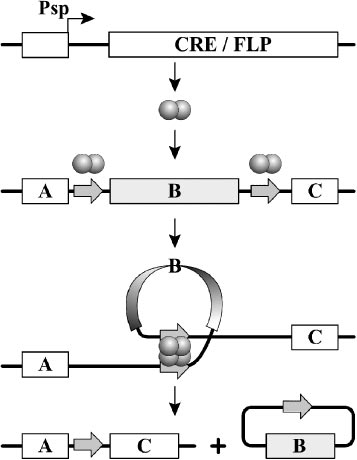
Controlling gene activity by site-specific recombination. Site-specific re-
combinases, usually Cre or Flp, are produced under the control of a tissue-specificpromoter, Psp. Dimeric recombinases interact with their specific binding sites (Lox orFRT), consisting of a 13-bp inverted repeat and an 8-bp asymmetric core sequence,which provides directionality. Association of two DNA-bound recombinase dimerscatalyzes a homologous recombination event within the 8-bp core sequence. Depend-ing on the orientation of the respective sites, this recombination event will either deleteor invert a "floxed" or "flrted" DNA fragment. Typically, a gene is knocked out by dele-tion of B provided it encodes an essential gene function. If B contains a stop sequenceseparating a regulatory region A from the functional gene C, deletion of B will placeC under the control of A and activate the C. In the usual binary approach, animals of aCre mouse line, for instance, are crossed with individuals of a floxed line. WheneverPsp becomes active in a double transgenic animal, recombination will occur in cellsspecified by Psp.
paradigm made use of repressor and operator of the lac operon in E. coli, whichwere shown to function in mammalian cell cultures (7, 27) and recently also intransgenic mice (13). Another prokaryotic repressor/operator system derived fromthe tet operon has been used in transgenic plants (21) and in mammalian tissuecultures (83). In these experimental designs where operator sequences are placedwithin an RNA polymerase II promoter, the operator-bound repressor appearsto sterically hinder the formation of transcription-competent RNA polymerase IIcomplexes. The action of the respective repressors is controlled at the level of DNAbinding by administering IPTG or Tc, respectively. To date, use of these repres-sion approaches has been limited. Why it appears more demanding to establish
CONDITIONAL GENE INACTIVATION
repression systems than activation systems (described below) has been discussedelsewhere (22).
The various transcription activation systems have one requirement in common:
"minimal" RNA polymerase II promoters. Ideally, such promoters are totally in-active by themselves whereas they are highly activated upon binding of artificialtranscription factors in their immediate vicinity. Depending upon the integrationsite within a genome, such conditions can indeed be established [discussed in(2, 17)]. The function of the respective transcription factors is subject to externalcontrol, which, in most cases, acts at the level of DNA binding. Examples for suchartificial transcription factors are fusion proteins where the DNA binding specificityis provided by, for example, the yeast Gal4 protein (79), the Drosophila ecdysonereceptor EcR (as heterodimer with the retenoid X receptor, RXR) (57), the repres-sors of the lac (37) or tet operon in E. coli. These DNA binding proteins are fusedto a transcription activation domain capable of stimulating RNA polymerase II.
Lac and Tet repressor fusions can be controlled by IPTG and Tc's, respectively,and EcR/RXR heterodimers are responsive to muristerone or ponasterone A. Bycontrast, as external control via carbohydrates is not feasible in mammalian cells,Gal4 fusions require the addition of a hormone binding domain, e.g., from theprogesterone receptor, that mediates regulation via mifepristone.
In a fundamentally different approach, split transcription factors were devel-
oped, with one moiety constituting the DNA binding domain, the other a transcrip-tional transactivation domain (55). Each of these domains is fused to a portion ofthe human FK506 binding protein that interacts with the drug. In the presenceof ligands like FK1012, a dimer of the immunosuppressant FK506, a functionaltranscription factor is constituted by dimerization of the two fusion proteins.
In principle, all these systems can be used to modulate gene activities, but
we focus on transcription activation systems that are controlled by tetracyclines,particularly by doxycycline (Dox), simply because these systems are by far themost widely used, not only at the level of cultured cells of plant, amphibian, insect,avian, and mammalian origin but also in whole organisms including S. cerevisiae(see below), Dictyostelium (5), Drosophila (4), plants, e.g., Arabidopsis (41), andmammals such as mice (see below). Despite this broad spectrum of applicationsand the exciting new insights into a variety of biological phenomena that manyhave yielded, we restrict this review primarily to the experimental model systemsoutlined in the introduction.
Controlling Transcription via Tetracyclines
The favorable properties of the Tet regulatory system as outlined in Figure 2 arebased on two crucial parameters: (a) the unusual specificity of interaction be-tween the Tet repressor (TetR) and its specific DNA binding site, the tet operator(tetO) and between Tet repressor and its inducer, particularly Dox, and (b) thewell-studied chemical and physiological properties of the inducing agents, i.e.,various tetracyclines. Some of these, particularly Dox, have been widely used inhuman and animal medicine for several decades. As outlined in Figure 2, there
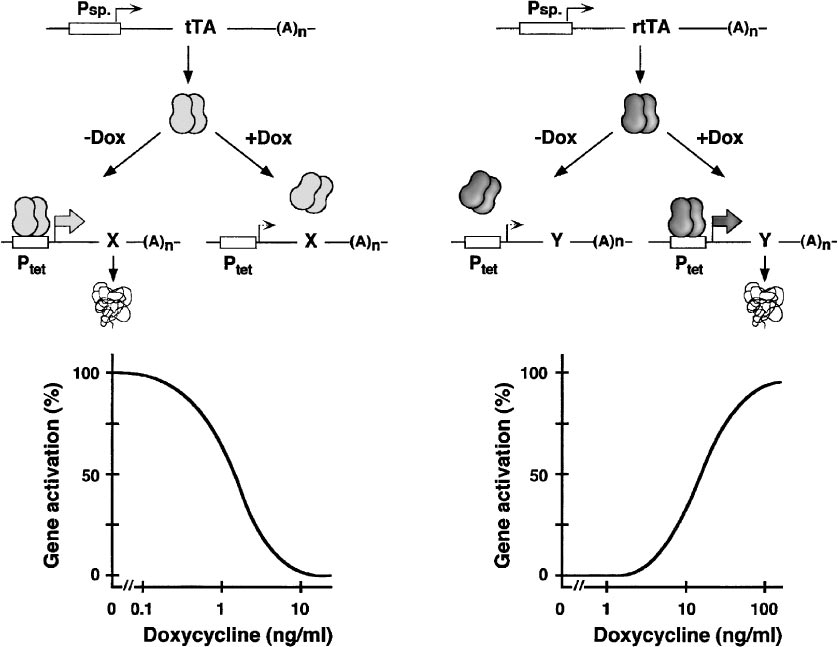
CONDITIONAL GENE INACTIVATION
are two complementary Tet control systems. In the tTA (tetracycline controlledtransactivator) or Tet-Off system, Dox prevents binding of tTA to Ptet, and thusabolishes transcription. By contrast, the rtTA (reverse tetracycline controlled trans-activator) or Tet-On system requires Dox for binding to and activation of Ptet(25). Although the various modifications and improvements of the Tet systemshave been described (2, 23), a few properties of the Tet systems should be noted.
Both systems can be set up in a way that allows extremely tight control and, atthe same time, regulation over a wide range, although to attain this quality ofcontrol is sometimes experimentally demanding (2, 17). To quantitatively controlexpression in cultured cells with either system, Dox concentrations are required(1 to 5 ng/ml for tTA, 50 to 100 ng/ml for rtTA-M2, see below) that are far be-low any toxicity threshold (5 to 10 µg/ml in HeLa cells). Due to the excellentcell and tissue penetration properties of Dox, these concentrations can be readilyachieved, even in different compartments of the mouse (34) including the pla-centa and the milk of lactating mothers (see below). Thus, Tet regulation canbe imposed on the developing embryo as well as on the offspring before andduring weaning. On the other hand, Dox penetrates the blood/brain barrier lessefficiently. Thus, the use of the rtTA system in the brain was problematic be-fore the recent advent of the M2 modifications (72). Finally, as Dox penetratescells by diffusion, a ridgeless regulation at the single cell level can be achievedwherever Dox concentration can be reliably titrated [see (35) and work describedbelow].
Outline of the Tet regulatory principle. Left upper part shows the mode of
action of the Tc-controlled transactivator (tTA). In the absence of the effector moleculeDox, tTA binds to the tetO sequence within Ptet and activates transcription of genex. Addition of Dox prevents tTA from binding and, thus, abolishes the initiation oftranscription. Left lower part depicts the dose response curve for the effects of Doxon tTA-dependent gene expression. Gene activity is maximal in the absence of theantibiotic but as effector concentrations increase, transcription gradually decreasesto background levels at Dox concentration ≥5 ng/ml. Right upper part illustrates themechanism of action of the reverse Tc-controlled transactivator (rtTA). rtTA is identicalto tTA except for 4 amino acid substitutions in the TetR moiety. rtTA requires Doxfor binding to tetO sequences within Ptet in order to activate transcription of gene y.
Right lower part outlines the dose response curve for the effects of Dox on the rtTA-dependent transcription activation. By increasing the effector concentration beyond20 ng/ml of Dox, rtTA-dependent gene expression is gradually stimulated. Ptet is aminimal promoter fused downstream of an array of 7 tet operators (24). It interactswith tTA as well as with rtTA. The new rtTA2S-M2 (72) is a highly improved versionof the original rtTA. It exhibits an enhanced sensitivity towards Dox and a negligibleresidual affinity towards tetO.
CONDITIONAL GENE ACTIVATION IN S. CEREVISIAE
The yeast Saccharomyces cerevisiae is an excellent model organism for the study ofgene function in eukaryotes, particularly as efficient homologous recombinationand reliable transcriptional induction systems can be exploited. Since the com-monly used transcription control systems such as the endogenous GAL promoterin combination with the inducer galactose cause pleiotropic effects, the Tet systemwas transferred to S. cerevisiae early on. Gari et al. (20) and Nagahashi et al. (47)were the first to successfully make this transfer.
Belli and colleagues (3) established a strategy whereby cellular promoters are
substituted by Ptet, placing the respective genes under Dox control. This versatileprinciple (63, 65) is now applied systematically as part of the EUROFAN projects(http://mips.gsf.de/proj/eurofan/; J. Hegemann, personal communication), whichanalyze a large number of essential yeast genes of unknown function. By system-atically substituting authentic promoters for Ptet, it is anticipated that insights intothe function of genes will be gained where null alleles or genomic sequences do notreveal direct clues to their physiological roles. The applicability of Tet regulationin fungal systems is underlined by the work of Nakayama and colleagues (48, 49),who transferred the essential regulatory elements into two Candida species where,in tTA-expressing strains, endogenous promoters were again replaced by Ptet, sub-jecting the respective genes to Tc control. Rather than using Candida as an eukary-otic model system, the focus of this work is to elucidate gene functions involvedin pathogenicity.
TARGETED CONDITIONAL MUTANTS OF
THE CHICKEN CELL LINE DT40
The chicken B cell line DT40 is distinct from other vertebrate cell lines in its abil-ity to promote extraordinary high rates of homologous chromosomal integrationupon introduction of suitable vector DNA (10). The frequency of recombinationby far exceeds even that observed in murine embryonic stem (ES) cells (14) andallows for the efficient sequential inactivation of all alleles (mostly two) of anygiven DT40 gene. Specific homologous recombination is carried out with clonedgenomic copies of the gene of interest, which is interrupted by an expression unitfor a selection marker. Upon transfer of the targeting vectors into DT40 cells,appropriate selection conditions yield a high percentage of stable clones, whichwill have one copy of the gene of interest inactivated by homologous recombina-tion. If this procedure is repeated with a different or, after recycling, even withthe same selection marker, the second genomic copy can be knocked out. Thisstrategy yields highly defined cell lines amenable to precise analysis. The experi-mental design is, however, limited to genes that are not essential for cell viability;it also lacks the advantage of reversible gene inactivation. This limitation has beenovercome by a conditionally active allele of the gene under investigation using the
CONDITIONAL GENE INACTIVATION
Tet system (11, 18, 19, 45, 46, 66–69, 75–78, 85). In most reports, when the firsttargeting round was performed as described, it was noted that the second rounddid not yield any homozygous knockout cells. To bypass this experimental bottle-neck, expression vectors containing the tTA gene under control of either Pcmv ora chicken beta-actin promoter were cotransfected with the gene of interest undercontrol of Ptet and tested for tetracycline-dependent expression. With the transgeneunit "on," the second endogenous gene copy could be targeted, thereby creatinga conditional knockout line. Two examples illustrate how such approaches aidthe phenotypic analysis of vertebrate gene function. Cleavage stimulation factorCstF is an evolutionarily conserved heterotrimeric protein factor involved in theprocessing of 30 ends of pre-mRNAs. In particular, in B-cells the expression ofIgM heavy chain genes switches via alternative splicing of the immunoglobin pre-mRNA from the membrane-bound form, as found in pre-B cells, to the secretedform, as found in plasma cells, presumably owing to increased concentrationsof CstF. Takagaki & Manley (68) directly addressed the function of CstF in thisprocess by placing the endogenous DT40 CstF-64 gene under control of the Tetsystem, according to the strategy described above. Not only did they find that thisgene is essential for viability, as depletion of its protein product causes apoptosis,but reduced concentrations of the proteins resulted in a reversible G0/G1 block ofthe cell cycle. More specifically, when CstF-64 concentrations were adjusted toabout 10% of the endogenous levels by varying the tetracycline concentration inthe medium, IgM expression, while greatly reduced on the overall mRNA level,was shifted toward the membrane-bound form of the immunoglobulin. This indi-cated a direct role of CstF concentration in the expression patterns of IgM heavychain in a developmentally regulated way. Another study aimed at unraveling therole of individual centromere proteins in chromosome segregation. Whereas thecentromere proteins CENP-A and CENP-C had been analyzed in some detail,a function for the more recently identified CENP-H remained to be established.
Fukagawa and colleagues (18) generated a conditional loss-of-function mutant ofDT40 CENP-H by introducing a Tet controlled transgene into these cells and sub-sequently inactivating the endogenous gene copy. As expected for a gene essentialfor centromere function, cells depleted of CENP-H arrested in metaphase. Con-comitant immunocyto-chemistry to localize CENPs with the transgene in either"on" or "off" state revealed that in the assembly of the centromere, CENP-A lo-calization is an early event, independent of CENP-H. By contrast, CENP-C couldnot be recruited to the centromere in CENP-H-depleted cells. Thus, these experi-ments established, in part, the order in which protein components assemble in theformation of a functional centromere.
Other studies were extended to reintroduce additional, modified copies of the
gene under investigation. While the conditional transgene was in the "off" state,this allowed, for example, for mutation and domain analyses (46, 69, 76, 78) as wellas interspecies complementation experiments (75). Evidence from phenotypic ex-amination in some studies provided direct support for Tc-regulated expression tooccur homogeneously at the single cell level in most if not all cells of a clonal
population [see, for example, Fukagawa et al. (19)]. The possibility of preciselytitrating intermediate rates of gene expression by varying the tetracycline con-centration in the tissue culture medium was demonstrated by Wang & Dreyfuss[(76), see also below]. Furthermore, conditional overexpression studies demon-strated that in the DT40 cell line the Tet system permits substantial (>100-fold)overexpression of transgenes when compared to rates of the endogenous genes(19).
Despite the exciting opportunities that this chicken cell line offers, so far only
a relatively small (but growing) community of researchers have taken advan-tage of it. However, DT40 cells will likely find more proponents in the futureas the potential of combining knockout technology with a reliable conditionalgene expression system becomes more obvious (9) and as the chicken genomeis sequenced (http://www.nih.gov/science/models/nmm/appd.html and referencestherein).
CONDITIONAL GENE ACTIVATION IN THE MOUSE
Transgenesis and ES cell technologies have opened up a unique access to themouse genome and converted this rodent into the most advanced model organismfor mammalian genetics. Predictably, approaches allowing for conditional mutage-nesis and for reversible gene activation/inactivation were introduced in this modelsystem early on, achieving an unprecedented degree of precision in the study ofin vivo gene function. Placing the cre gene under control of defined tissue-specificpromoters limits the recombination event to defined stages of the animal's de-velopment and to specific tissues, frequently preventing embryonic lethality ordevelopmental adaptation. The impact of this approach is reflected by the "zoo"of mouse lines that either express the cre gene under the control of various tissue-specific promoters or contain a variety of genes equipped with Lox sites for geneinactivation, activation, or alteration (for information on such mouse lines, seehttp://www.mshri.on.ca/nagy/cre.htm). The synergism generated by the numberof mouse lines available is rapidly increasing the impact of this technology. On theother hand, Cre-induced recombination results in irreversible genetic alterationsthat reflect the temporal and spatial activity spectrum, i.e., the activity "history"of the promoter driving the cre gene. Thus, unless Cre activity is controlled fromoutside, this approach is limited not only by the irreversibility but also by the rigid-ity of the developmental and differentiation program of the organism, which, forexample, prevents recombination in a fully differentiated tissue at a later periodin the animal's life. In principle, these limitations can be overcome by systemsthat allow gene expression to be controlled from outside at will. As noted, thereare excellent reviews on the achievements of the Cre/Lox and the Flp/FRT systemin the mouse. We therefore focus here on applications of the Tet regulatory sys-tems that particularly demonstrate the power of reversible gene inactivation. Wealso discuss approaches for controlling recombinase activities from outside andpoint out the beginning synergism generated by combining the Cre/Lox with the
CONDITIONAL GENE INACTIVATION
Tet system by making use of the collection of transgenic mouse lines from bothworlds.
Controlling Dominant Negative Gene Products
The most straightforward approach for studying gene functions in a transgenicmouse uses a dominant negative mutation version of the gene of interest placedunder the control of the Tet system. When activated at the desired time pointwithin the life span of the animal, an altered phenotype is expected that may beabrogated when the expression of the mutant gene is switched off again. Tissue- orcell type–specificity is achieved by placing the tTA/rtTA gene under control of theappropriate promoter. Impressive first examples of this approach were describedby Kandel's group (42, 43); they generated mouse lines expressing tTA or rtTA, re-spectively, under the control of the αCamKII promoter that restricts the presence oftTA/rtTA to defined regions of the forebrain, particularly the hippocampus. Cross-ing these mice with animals containing dominant negative versions of αCamKIIor calcineurin, respectively, yielded animals suitable for the study of synaptic plas-ticity in the context of learning and memory formation. Thus, animals could notmaster a spatial learning task when, for example, the dominant negative versionof the αCamKII gene was active (43). The full potential of conditional expressionwas revealed when the mice were put through the learning procedure before thedominant negative genes were activated, for activation resulted in loss of memory.
Most interestingly, however, when switched back to the wild-type situation bysupplying Dox in the drinking water, spatial memory returned. This indicates thatαCamKII is required not only for learning and memory formation but also for theretrieval of information from memory.
Today, more than 40 mouse lines have been published where tTA or rtTA is
controlled by a variety of promoters, and about the same number of lines con-taining various genes and mutant versions of genes under Ptet control have beendescribed [for review see (60)]. Among the many exciting findings are some wherereversibility of gene activation was a prerequisite for gaining new insights into tu-mor pathology. Several oncogenes, including H-RasV12G (12), K-Ras4bG12D (16),c-myc (15), ErbB2 (81), and BCR-ABL-1 (28), have been placed under Tet con-trol. In all cases, malignant tumors could be induced in specific tissues in theadult animal. Unexpectedly, however, in all systems the vast majority of tumorsremained fully dependent on the continued expression of the tumor-initiating onco-gene, as demonstrated by the complete regression of tumors upon inactivation ofthe respective oncogene. In another exciting result, Yamamoto et al. (82) reportedthat activation of a mutant huntingtin gene in the brain of adult mice inducedmassive anatomical and neurological symptoms in the animals. Upon inactiva-tion of the mutant huntingtin gene by Dox, the deposits in the animal's braindissolved and the animals appeared to fully recover. These are the first results toindicate that Huntington disease may be reversible even after the manifestationof massive symptoms, provided that the activity of the huntingtin gene can beabrogated.
Surviving Development by Conditional Transgene Expression
There are numerous well-documented examples for embryonic or neonatal phe-notypes in mice after gene knockouts or after transgene over- or misexpressionduring early development. In gene knockouts, the time of death only reflects thefirst stage of development where a particular gene is indispensable when no re-dundant gene activity is available for compensation. Even though this might beinformative, embryonic death is rarely the result hoped for when analysis of thetargeted gene was envisaged. In biomedical research, to name just one example,most questions addressed to an animal model require adulthood. Obviously, con-ditionality in transgene expression could overcome these limitations, provided thatthe systems warrant exogenous experimental control in pre- and perinatal devel-opment. In the case of the Tet system, this would require maternal delivery of Doxin utero and via breast milk (38, 53). Several groups (53) have demonstrated that,as expected, Dox fulfills these conditions. By regulating expression rates of IL-11in the embryonic, neonatal, and adult lung, Ray and colleagues could discriminatebetween development-dependent and -independent phenotypic consequences ofIL-11 overexpression on lung growth and morphology. A second example is theconditional expression of the receptor tyrosine kinase ErbB2 in transgenic mice(81). Expression of this oncogene in epithelial cells via the keratin 14 (K14) pro-moter resulted in hyperplasia and abnormal hair follicle morphogenesis but deathof the animals around birth prevented the analysis of ErbB2 in adult mice. However,when the K14 promoter was used to drive expression of rtTA and ErbB2 was placedunder the control of Ptet, adult transgenic animals were obtained and controlledexpression via Dox administration established a direct link between overexpres-sion of ErbB2 and skin hyperplasia, which was reversible upon withdrawal of theinducing substance. Meanwhile, there are many examples of transgenic mouselines where embryonic Tet control over transgenes prevented an otherwise lethaloutcome of the experiments [see (28, 31, 54)].
Homologous recombination as used for the generation of knockout mice provedto be an indispensable tool for the analysis of gene function in mammals. In its"simplest" version, this technology is used to inactivate one copy of an endogenousgene by recombination with a suitable targeting construct at the level of ES cells.
Subsequent breeding of ES cell-derived mice to homozygosity with respect to thetargeted gene then leads to the desired genotype. Using this basic approach, it isalso possible to modify, rather than to inactivate, an endogenous gene by substitut-ing parts or the entire gene with sequences of differing function. Such "knock-ins"provide unparalleled possibilities in dissecting the function of an individual genein vivo, but again potential embryonic lethality or compensatory development lim-its this approach. Early studies now show that these limitations can be overcomeby combining conditional gene expression with knock-in approaches. Thus, Tilgh-man and coworkers (62) analyzed the temporal requirements for expression of the
CONDITIONAL GENE INACTIVATION
Ednrb, the gene for the endothelin receptor B in murine development. Homozygousnull mutant mice for this gene die as juveniles. Shin et al. (62) created two differentknock-in lines, the first by substituting Ednrb for either tTA or rtTA. This strategyensures that the spatial and temporal expression patterns of the transactivators mostlikely follow that of the targeted gene while inactivating one copy of that gene. Inthe second line, the promoter of Ednrb was substituted for Ptet-1, which also re-sulted in the inactivation of that gene copy. Crosses between these two lines madeexpression of Ednrb conditionally dependent on Dox. Reverting the functionalnull mutation during defined time windows revealed that Ednrb is only requiredbetween days 10 and 12.5 of embryonic development. In another study, Adelmanand coworkers (6) analyzed a gene for one of the subunits of calcium-activatedpotassium channels, SK3. Here, only one knock-in targeting construct was usedto bring tTA under control of the endogenous SK3 promoter and to simultane-ously place Ptet-1 in front of the endogenous gene. When the respective mice werebred to homozygosity, the functional null mutation (induced by administration ofDox) did not cause a distinct phenotype. By contrast, overexpression of SK3 in theabsence of Dox clearly demonstrated a role of SK3 in respiratory patterns underhypoxic conditions. Not only did that study exemplify the tight control that theTet system can exert over endogenous genes, it also demonstrated that expressionrates for endogenous genes can be achieved well in excess of the natural situation.
Yet another strategy was followed in an attempt to model Charcot-Marie-Tooth
disease in transgenic mice, a demyelinating neuropathy believed to be a result ofoverexpression of peripheral myelin protein 22 (PMP22). Previous studies hadshown that a yeast artificial chromosome (YAC) encompassing the region of thatgene faithfully reflects the expression patterns of PMP22 in mice. Perea et al. (52)therefore chose such a YAC and placed tTA under control of the PMP22 promoter.
Upon transgenesis, tTA was expressed as expected, and when respective animalswere crossed with transgenic mice harboring the PMP22 gene under control of Ptet,the resulting double transgenic mice allowed the time course of demyelination andthe development of characteristic pathology upon overexpression of PMP22 tobe followed. Again, by downmodulating PMP22 expression via administration ofDox, demyelination and pathology were corrected.
As indicated above, controlling the activity of recombinases in vivo would addprecision and another degree of freedom to the conditional knockout approachesvia site-specific recombination. Besides controlling cre or flp gene expression, e.g.,by the Tet system (see below), modified recombinases were developed wherebyactivity can be modulated directly by respective effector molecules. By fusing Creto mutated ligand binding domains (LBD) of the progesterone (32) or estrogen(44) receptor, transport of the fusion protein and thus of the enzymatic activity inthe cell nucleus can be controlled by the synthetic steroid Ru486 or tamoxifen, re-spectively. High recombination efficiencies were obtained in the mouse skin (74)and in adipocytes (29), for example, by using a Cre-ER fusion and tamoxifen.
Two experimental parameters initially limited the Cre-ER-tamoxifen approach:the high, almost toxic concentrations of inducer required and the residual recom-binase activity in the absence of the inducer. The first problem has apparentlybeen solved by generating novel fusions between Cre and LBDs of the proges-terone (80) and estrogen receptors (30), with enhanced sensitivities towards theirrespective ligands. However, the tightness of regulation still poses a problem, par-ticularly when targeting cell types with a long lifetime, such as most neuronal cells.
Thus, in the respective tissues, cells will accumulate over time that have undergoneCre-dependent deletions, and even at low recombination rates a phenotype mayeventually be seen in the uninduced state. On the other hand, in many tissues witha high turnover of cells such as skin, hormone-controlled recombinases appear tobe suitable tools.
An obvious alternative approach is to control the expression of recombinases.
Even though this strategy may be experimentally demanding, there are exampleswhere the Tet system, for instance, was used to efficiently control cre gene ex-pression. Thus, Lee and coworkers (73) generated mouse lines in which the rtTAgene, controlled by the retinoblastoma or the whey acidic promoter and the Ptet-Cre expression unit, were transferred as a single construct. By breeding animals ofthese lines with mice containing floxed genes, they demonstrated accurate controlof recombination via Dox. In an interesting though rather complex variation ofthat theme, Tsien and coworkers (61) generated a mouse line where the tTA geneis placed under control of an ubiquitous β-actin promoter, separated, however, bya floxed stop-sequence, which rendered tTA expression sensitive to Cre action.
Thus, whenever Cre is produced under the control of a tissue-specific promoter,the block of tTA expression is removed in the respective cell type and expressionof the Ptet-controlled transgene becomes Dox dependent. As in these experimentsfloxed copies of an endogenous gene were deleted simultaneously with the stop-sequence of the tTA gene, this particular experimental design seems highly, if notprohibitively, demanding with respect to the breeding efforts required. However,simplified versions of this approach can be envisaged, as already indicated by thework of Utomo et al. (73).
As Tet control can be set up in mice such that there is very tight regulation of Cre
(K. Sch¨onig, F. Schwenk, K. Rajewsky & H. Bujard, in preparation), respectivemouse lines would allow two sizable collections of transgenics to be exploited:mouse lines that express tTA or rtTA tissue specifically, and mouse lines carryingvarious floxed genes. Even though this approach will require significant breedingefforts, it allows many questions to be addressed without the need for generatingan additional transgenic mouse line.
In recent years, application of the methodological principles discussed here hasadded considerably to our understanding of gene function in vivo and is begin-ning to provide fundamental insights into such complex biological phenomenaas development, behavior, and disease. The impact of conditional gene activation/
CONDITIONAL GENE INACTIVATION
inactivation approaches is likely to increase as the various experimental systems be-come more sophisticated and are adapted to further model organisms. At the sametime, consideration of the intricate action of genes in vivo reveals both the strengthand the limitations of current experimental capabilities. For example, many genesare active within distinct time windows and in different cell types or tissues duringdevelopment and adulthood of an organism. Their proper function also dependson the rate of expression, which in turn may be influenced by environmental sig-nals. Accordingly, there are many examples showing that misexpression of a gene,i.e., over- or underexpression or expression outside the genuine developmental ordifferentiation program, results in pathologies. Mimicking such misexpression intransgenic mice has led to numerous disease models that are of particular interestwhenever the disease-causing gene activity can be controlled from outside. Impor-tant questions concerning the onset, the progression, and particularly the potentialreversibility of a disease can thus be addressed. As discussed above, several mousemodels were generated whereby the expression of oncogenes could be controlledfrom outside. In all model systems, malignant tumors were induced that, consid-ering the lag time between oncogene induction and invasive tumor growth, appearto have accumulated additional mutations. This is in agreement with the prevailinghypothesis that multiple genetic changes are required for persistent tumor growth(33). Surprisingly, upon inactivation of the tumor-initiating gene, the overwhelm-ing number of tumors regressed, demonstrating that the respective oncogenes arealso required for tumor maintenance. Moreover, mice that recovered from such tu-mors and were kept under the respective conditions had a normal life expectancy.
As tumor regression in these systems is largely due to apoptosis induced by the shut-off of the initiating oncogene, it appears that the tumor cells had adapted to a rela-tively rigid oncogene-dependent expression program that cannot be reverted quick-ly enough to prevent activation of apoptosis (16). These findings not only point toand validate promising drug targets, they also challenge some generally acceptedviews on tumor development and persistence. There are further reports where dis-ease models based on the above strategy uncovered unexpected mechanisms ofdisease development and regression [e.g. (71, 82)]. Together with the exciting workof Kandel's group on the mechanisms of brain plasticity and memory (see above),these reports exemplify the power of genetic analysis by reversible perturbationsthat may even be induced and released repeatedly during the life span of an animal.
A major experimental challenge is the controlled perturbation of expression
rates to examine genes involved in regulatory networks, for example. Generally,the products of such genes interact with multiple partners at different affinitiesand, thus, minor variations in intracellular equilibria are likely to invoke distinctphenotypes. A. Smith's group (50), in an interesting example, showed in mouseES cells that by titrating the expression of transcription factor Oct3/4 within asmall margin, three distinct cell fates can be induced. Thus, it appears likely that byvarying intracellular concentrations of gene products, a large number of phenotypesobtained by knockout strategies will separate into different phenotypes, indicatingthat the respective gene product participates in multiple equilibria and possiblyreveals novel interaction partners.
Although expression rates can be readily fine-tuned in cultured cells or uni-
cellular organisms, such fine-tuning in transgenic mice poses an experimentalchallenge. Nevertheless, it may well be feasible to some extent, provided there isfavorable information on tissue penetration and distribution, biological half-lifetime, etc., of the inducing agent. Indeed, by coregulating a marker gene whoseactivity can be monitored noninvasively, the activity of a gene of interest can befollowed over long periods of time and adjusted to desired rates (26).
Despite the great potential of the various experimental approaches available to-
day, many problems remain. Thus, as long as we know little about the influence ofchromatin structure on gene expression and its sensitivity to perturbation, particu-larly when modifying regulatory regions, reliable experimental designs where celltype specificity is maintained and position effect variegation prevented will remaindifficult, if not impossible. Precise and minimal disturbance gene targeting and theapplication of the BAC technology will certainly be most valuable in future. Onthe other hand, approaches that leave the gene of interest fully untouched in itsgenomic context may be available soon. For example, controlling custom-made,zinc finger–based transcription factors recognizing specific sequences within thepromoter of the gene of interest or regulated synthesis of RNAs or polypeptides(including modified antibodies) that attack specific gene products may developinto systems of choice. Finally, despite the rapid kinetics by which, for example,Tet-controlled transcription can be induced (<5 min in cultured cells, <1 h inthe liver of transgenic mice), many biological processes are governed by vastlyfaster mechanisms that can hardly be addressed by methods currently available.
Obviously, in our strivings toward a better understanding of in vivo gene function,there are no limits for new ideas.
The authors thank Drs. Johannes Hegemann and G¨unther Sch¨utz for helpful sci-entific discussions. Ms Sibylle Reinig is recognized for help in the preparation ofthis manuscript. Work in the authors' laboratories is supported by the Volkswa-genStiftung (program: "Conditional Mutagenesis," grants to M.G. and H.B.), anEC-grant (H.B.), and the Fonds der Chemischen Industrie Deutschlands (H.B.).
The Annual Review of Genetics is online at http://genet.annualreviews.org
1. Abuin A, Bradley A. 1996. Recycling
3. Belli G, Gari E, Aldea M, Herrero E.
selectable markers in mouse embryonic
1998. Functional analysis of yeast essen-
stem cells. Mol. Cell Biol. 16:1851–56
tial genes using a promoter-substitution
2. Baron U, Bujard H. 2000. Tet repressor-
cassette and the tetracycline-regulatable
based system for regulated gene expres-
dual expression system. Yeast 14:1127–
sion in eukaryotic cells: principles and ad-
vances. Methods Enzymol. 327:401–21
4. Bello B, Resendez-Perez D, Gehring WJ.
CONDITIONAL GENE INACTIVATION
1998. Spatial and temporal targeting of
studies: experimental evidence in support
gene expression in Drosophila by means
of chicken DT40 cell line as a unique
of a tetracycline-dependent transactivator
model. J. Environ. Pathol. Toxicol. Oncol.
system. Development 125:2193–202
5. Blaauw M, Linskens MH, van Haastert PJ.
15. Felsher DW, Bishop JM. 1999. Reversible
2000. Efficient control of gene expression
tumorigenesis by MYC in hematopoietic
by a tetracycline-dependent transactivator
lineages. Mol. Cell 4:199–207
in single Dictyostelium discoideum cells.
16. Fisher GH, Wellen SL, Klimstra D, Lenc-
Gene 252:71–82
zowski JM, Tichelaar JW, et al. 2001. In-
6. Bond CT, Sprengel R, Bissonnette JM,
duction and apoptotic regression of lung
Kaufmann WA, Pribnow D, et al. 2000.
adenocarcinomas by regulation of a K-
Respiration and parturition affected by
Ras transgene in the presence and absence
conditional overexpression of the Ca2+-
of tumor suppressor genes. Genes Dev. 15:
activated K+ channel subunit, SK3. Sci-
17. Freundlieb S, Schirra-Muller C, Bujard
7. Brown M, Figge J, Hansen U, Wright C,
H. 1999. A tetracycline controlled activa-
Jeang KT, et al. 1987. lac repressor can
tion/repression system with increased po-
regulate expression from a hybrid SV40
tential for gene transfer into mammalian
early promoter containing a lac operator
cells. J. Gene Med. 1:4–12
in animal cells. Cell 49:603–12
18. Fukagawa T, Mikami Y, Nishihashi A,
8. Buchholz F, Angrand PO, Stewart AF.
Regnier V, Haraguchi T, et al. 2001.
1998. Improved properties of FLP recom-
CENP-H, a constitutive centromere com-
binase evolved by cycling mutagenesis.
ponent, is required for centromere target-
Nat. Biotechnol. 16:657–62
ing of CENP-C in vertebrate cells. EMBO
9. Buerstedde JM, Arakawa H, Watahiki A,
J. 20:4603–17
Carninci PP, Hayashizaki YY, et al. 2002.
19. Fukagawa T, Pendon C, Morris J, Brown
The DT40 web site: sampling and con-
W. 1999. CENP-C is necessary but not
necting the genes of a B cell line. Nucleic
sufficient to induce formation of a func-
Acids Res. 30:230–31
tional centromere. EMBO J. 18:4196–209
10. Buerstedde JM, Takeda S. 1991. Increased
20. Gari E, Piedrafita L, Aldea M, Herrero E.
ratio of targeted to random integration af-
1997. A set of vectors with a tetracycline-
ter transfection of chicken B cell lines.
regulatable promoter system for modu-
Cell 67:179–88
lated gene expression in Saccharomyces
11. Chen Z, Manley JL. 2000. Robust mRNA
cerevisiae. Yeast 13:837–48
transcription in chicken DT40 cells de-
21. Gatz C, Frohberg C, Wendenburg R. 1992.
pleted of TAF(II)31 suggests both func-
Stringent repression and homogeneous
tional degeneracy and evolutionary diver-
derepression by tetracycline of a modi-
gence. Mol. Cell Biol. 20:5064–76
fied CaMV 35S promoter in intact trans-
12. Chin L, Tam A, Pomerantz J, Wong M,
genic tobacco plants. Plant J. 2:397–
Holash J, et al. 1999. Essential role for
oncogenic Ras in tumour maintenance.
22. Gossen M, Bonin AL, Bujard H. 1993.
Control of gene activity in higher eukary-
13. Cronin CA, Gluba W, Scrable H. 2001.
otic cells by prokaryotic regulatory ele-
The lac operator-repressor system is func-
ments. Trends Biochem. Sci. 18:471–75
tional in the mouse. Genes Dev. 15:1506–
23. Gossen M, Bujard H. 2001. Tetracy-
clines in the control of gene expression
14. Dhar PK, Sonoda E, Fujimori A, Ya-
in eukaryotes. In Tetracyclines in Biology,
mashita YM, Takeda S. 2001. DNA repair
Chemistry and Medicine, ed. M Nelson,
W Hillen, RA Greenwald, pp. 139–57.
from hereditary colorectal cancer. Cell
Basel: Birkh¨auser Verlag
24. Gossen M, Bujard H. 1992. Tight control
34. Kistner A, Gossen M, Zimmermann
of gene expression in mammalian cells by
F, Jerecic J, Ullmer C, et al. 1996.
tetracycline-responsive promoters. Proc.
Doxycycline-mediated quantitative and
Natl. Acad. Sci. USA 89:5547–51
tissue-specific control of gene expression
25. Gossen M, Freundlieb S, Bender G,
in transgenic mice. Proc. Natl. Acad. Sci.
Muller G, Hillen W, et al. 1995. Trans-
USA 93:10933–38
criptional activation by tetracyclines in
35. Kringstein AM, Rossi FMV, Hofmann A,
mammalian cells. Science 268:1766–69
Blau HM. 1998. Graded transcriptional
26. Hasan MT, Schonig K, Berger S, Graewe
response to different concentrations of a
W, Bujard H. 2001. Long-term, noninva-
single transactivator. Proc. Natl. Acad.
sive imaging of regulated gene expression
Sci. USA 95:13670–75
in living mice. Genesis 29:116–22
36. K¨uhn R, Schwenk F. 2002. Conditional
27. Hu MC, Davidson N. 1987. The inducible
knockout mice. See Ref. 73a, pp. 159–86
lac operator-repressor system is func-
37. Labow MA. 1995. Use of lac activator
tional in mammalian cells. Cell 48:555–
proteins for regulated expression of onco-
genes. Methods Enzymol. 254:375–87
28. Huettner CS, Zhang P, Van Etten RA,
38. Lee P, Morley G, Huang Q, Fischer A,
Tenen DG. 2000. Reversibility of acute
Seiler S, et al. 1998. Conditional lineage
B-cell leukaemia induced by BCR-ABL1.
ablation to model human diseases. Proc.
Nat. Genet. 24:57–60
Natl. Acad. Sci. USA 95:11371–76
29. Imai T, Jiang M, Chambon P, Metzger D.
39. Lewandoski M. 2001. Conditional control
2001. Impaired adipogenesis and lipolysis
of gene expression in the mouse. Nat. Rev.
in the mouse upon selective ablation of the
Genet. 2:743–55
retinoid X receptor alpha mediated by a
40. Loonstra A, Vooijs M, Beverloo HB, Al-
tamoxifen-inducible chimeric Cre recom-
lak BA, van Drunen E, et al. 2001. Growth
binase (Cre-ERT2) in adipocytes. Proc.
inhibition and DNA damage induced by
Natl. Acad. Sci. USA 98:224–28
Cre recombinase in mammalian cells.
30. Indra AK, Warot X, Brocard J, Bornert
Proc. Natl. Acad. Sci. USA 98:9209–14
JM, Xiao JH, et al. 1999. Temporally-
41. Love J, Scott AC, Thompson WF. 2000.
controlled site-specific mutagenesis in the
basal layer of the epidermis: compari-
of transgene expression in Arabidopsis
son of the recombinase activity of the
thaliana using the Top10 promoter sys-
tamoxifen-inducible Cre-ER(T) and Cre-
tem. Plant J. 21:579–88
ER(T2) recombinases. Nucleic Acids Res.
42. Mansuy IM, Winder DG, Moallem TM,
Osman M, Mayford M, et al. 1998. In-
31. Jerecic J, Schulze CH, Jonas P, Spren-
ducible and reversible gene expression
gel R, Seeburg PH, et al. 2001. Im-
with the rtTA system for the study of mem-
paired NMDA receptor function in mouse
ory. Neuron 21:257–65
olfactory bulb neurons by tetracycline-
43. Mayford M, Bach ME, Huang YY, Wang
sensitive NR1 (N598R) expression. Brain
L, Hawkins RD, et al. 1996. Control of
Res. Mol. Brain Res. 94:96–104
memory formation through regulated ex-
32. Kellendonk C, Tronche F, Casanova E,
pression of a CaMKII transgene. Science
Anlag K, Opherk C, et al. 1999. Inducible
site-specific recombination in the brain. J.
44. Metzger D, Clifford J, Chiba H, Cham-
Mol. Biol. 285:175–82
bon P. 1995. Conditional site-specific re-
33. Kinzler KW, Vogelstein B. 1996. Lessons
combination in mammalian cells using a
CONDITIONAL GENE INACTIVATION
ligand-dependent chimeric Cre recombi-
to dissociate development-dependent and
nase. Proc. Natl. Acad. Sci. USA 92:6991–
-independent phenotypes. J. Clin. Invest.
45. Morrison C, Shinohara A, Sonoda E,
54. Rhoades KL, Hetherington CJ, Harakawa
Yamaguchi-Iwai Y, Takata M, et al. 1999.
N, Yergeau DA, Zhou L, et al. 2000.
The essential functions of human Rad51
Analysis of the role of AML1-ETO in
are independent of ATP hydrolysis. Mol.
leukemogenesis, using an inducible trans-
Cell Biol. 19:6891–97
genic mouse model. Blood 96:2108–
46. Morrison C, Sonoda E, Takao N, Shino-
hara A, Yamamoto K, et al. 2000. The
55. Rivera VM. 1998. Controlling gene ex-
controlling role of ATM in homologous
pression using synthetic ligands. Methods
recombinational repair of DNA dam-
age. EMBO J. 19:463–71. Erratum. 2000.
56. Ryding AD, Sharp MG, Mullins JJ. 2001.
EMBO J. 19(4):786
Conditional transgenic technologies. J.
47. Nagahashi S, Nakayama H, Hamada K,
Yang H, Arisawa M, et al. 1997. Regu-
57. Saez E, No D, West A, Evans RM. 1997.
lation by tetracycline of gene expression
Inducible gene expression in mammalian
in Saccharomyces cerevisiae. Mol. Gen.
cells and transgenic mice. Curr. Opin.
48. Nakayama H, Izuta M, Nagahashi S,
58. Sauer B, Henderson N. 1989. Cre-stim-
Sihta EY, Sato Y, et al. 1998. A con-
ulated recombination at loxP-contain-
trollable gene-expression system for the
ing DNA sequences placed into the mam-
pathogenic fungus Candida glabrata. Mi-
malian genome. Nucleic Acids Res. 17:
49. Nakayama H, Mio T, Nagahashi S, Ko-
59. Schmidt EE, Taylor DS, Prigge JR, Bar-
kado M, Arisawa M, et al. 2000. Tetra-
nett S, Capecchi MR. 2000. Illegitimate
cycline-regulatable system to tightly con-
Cre-dependent chromosome rearrange-
trol gene expression in the pathogenic
ments in transgenic mouse spermatids.
fungus Candida albicans. Infect. Immun.
Proc. Natl. Acad. Sci. USA 97:13702–7
60. Sch¨onig K, Bujard H. 2002. Generating
50. Niwa H, Miyazaki J, Smith AG. 2000.
conditional mouse mutants via tetracy-
Quantitative expression of Oct-3/4 defines
cline controlled gene expression. See Ref.
differentiation, dedifferentiation or self-
73a, pp. 69–104
renewal of ES cells. Nat. Genet. 24:372–
61. Shimizu E, Tang Y-P, Rampon C, Tsien
JZ. 2000. NMDA receptor-dependent syn-
51. O'Gorman S, Fox DT, Wahl GM. 1991.
aptic reinforcement as a crucial process
Recombinase-mediated gene activation
for memory consolidation. Science 290:
and site-specific integration in mammal-
ian cells. Science 251:1351–55
62. Shin MK, Levorse JM, Ingram RS, Tilgh-
52. Perea J, Robertson A, Tolmachova T,
man SM. 1999. The temporal require-
Muddle J, King RH, et al. 2001. Induced
ment for endothelin receptor-B signalling
myelination and demyelination in a con-
during neural crest development. Nature
ditional mouse model of Charcot-Marie-
Tooth disease type 1A. Hum. Mol. Genet.
63. Shirra MK, Patton-Vogt J, Ulrich A,
Liuta-Tehlivets O, Kohlwein SD, et al.
53. Ray P, Tang W, Wang P, Homer R, Kuhn
2001. Inhibition of acetyl coenzyme A
C, et al. 1997. Regulated overexpres-
carboxylase activity restores expression
sion of Interleukin 11 in the lung. Use
of the INO1 gene in a snf1 mutant strain
of Saccharomyces cerevisiae. Mol. Cell.
dent transcriptional activators: novel mu-
tations yield expanded range and sensitiv-
64. Silver DP, Livingston DM. 2001. Self-
ity. Proc. Natl. Acad. Sci. USA 97:7963–
excising retroviral vectors encoding the
Cre recombinase overcome Cre-mediated
73. Utomo AR, Nikitin AY, Lee WH. 1999.
cellular toxicity. Mol. Cell 8:233–43
Temporal, spatial, and cell type-specific
65. Simon E, Clotet J, Calero F, Ramos
control of Cre-mediated DNA recombina-
J, Arino J. 2001. A screening for high
tion in transgenic mice. Nat. Biotechnol.
copy suppressors of the sit4 hal3 syn-
thetically lethal phenotype reveals a role
73a. van Deursen J, Hofker M, eds. 2002. The
for the yeast Nha1 antiporter in cell cy-
Transgenic Mouse—Methods and Proto-
cle regulation. J. Biol. Chem. 276:29740–
cols. Totowa, NJ: Humana
74. Vasioukhin V, Degenstein L, Wise B,
66. Sonoda E, Sasaki MS, Buerstedde JM,
Fuchs E. 1999. The magical touch:
Bezzubova O, Shinohara A, et al. 1998.
genome targeting in epidermal stem cells
Rad51-deficient vertebrate cells accumu-
induced by tamoxifen application to
late chromosomal breaks prior to cell
mouse skin. Proc. Natl. Acad. Sci. USA
death. EMBO J. 17:598–608
67. Sonoda E, Sasaki MS, Morrison C,
75. Wang J, Dreyfuss G. 2001. A cell system
Yamaguchi-Iwai Y, Takata M, et al. 1999.
with targeted disruption of the SMN gene.
Sister chromatid exchanges are mediated
Functional conservation of the SMN pro-
by homologous recombination in ver-
tein and dependence of gemin2 on SMN.
tebrate cells. Mol. Cell Biol. 19:5166–
J. Biol. Chem. 276:9599–605
76. Wang J, Dreyfuss G. 2001. Characteriza-
68. Takagaki Y, Manley JL. 1998. Levels of
tion of functional domains of the SMN
polyadenylation factor CstF-64 control
protein in vivo. J. Biol. Chem. 276:45387–
IgM heavy chain mRNA accumulation
and other events associated with B cell
77. Wang J, Takagaki Y, Manley JL. 1996.
differentiation. Mol. Cell 2:761–71
Targeted disruption of an essential verte-
69. Takami Y, Nakayama T. 2000. N-terminal
brate gene: ASF/SF2 is required for cell
region, C-terminal region, nuclear export
viability. Genes Dev. 10:2588–99
signal, and deacetylation activity of his-
78. Wang J, Xiao SH, Manley JL. 1998. Ge-
tone deacetylase-3 are essential for the vi-
netic analysis of the SR protein ASF/SF2:
ability of the DT40 chicken B cell line. J.
interchangeability of RS domains and
Biol. Chem. 275:16191–201
negative control of splicing. Genes Dev.
70. Thyagarajan B, Guimaraes MJ, Groth AC,
Calos MP. 2000. Mammalian genomes
79. Wang Y, Tsai SY, O'Malley BW. 1999.
contain active recombinase recognition
Antiprogestin regulable gene switch for
sites. Gene 244:47–54
induction of gene expression in vivo.
71. Tremblay P, Meiner Z, Galou M, Heinrich
Methods Enzymol. 306:281–94
C, Petromilli C, et al. 1998. Doxycycline
80. Wunderlich FT, Wildner H, Rajewsky K,
control of prion protein transgene expres-
Edenhofer F. 2001. New variants of in-
sion modulates prion disease in mice.
ducible Cre recombinase: a novel mu-
Proc. Natl. Acad. Sci. USA 95:12580–
tant of Cre-PR fusion protein exhibits en-
hanced sensitivity and an expanded range
72. Urlinger S, Baron U, Thellmann M, Hasan
of inducibility. Nucleic Acids Res. 29:E47
MT, Bujard H, et al. 2000. Exploring the
81. Xie W, Chow LT, Paterson AJ, Chin E,
sequence space for tetracycline-depen-
Kudlow JE. 1999. Conditional expression
CONDITIONAL GENE INACTIVATION
of the ErbB2 oncogene elicits reversible
sor, tetR, rather than the tetR-mammalian
hyperplasia in stratified epithelia and
cell transcription factor fusion derivatives,
up-regulation of TGFalpha expression
regulates inducible gene expression in
in transgenic mice. Oncogene 18:3593–
mammalian cells. Hum. Gene Ther. 9:
82. Yamamoto A, Lucas JJ, Hen R. 2000. Re-
84. Yu Y, Bradley A. 2001. Engineering chro-
versal of neuropathology and motor dys-
mosomal rearrangements in mice. Nat.
function in a conditional model of Hunt-
Rev. Genet. 2:780–90
ington's disease. Cell 101:57–66
85. Zhao W, Manley JL. 1998. Deregulation
83. Yao F, Svensjo T, Winkler T, Lu M, Eriks-
of poly(A) polymerase interferes with cell
son C, et al. 1998. Tetracycline repres-
growth. Mol. Cell Biol. 18:5010–20
Source: http://biologie.univ-mrs.fr/upload/p102/annurev.genet.36.041002.120114.pdf
Comment on the draft terms of reference (TOR)—Aquis Resort at the Great Barrier Reef project Comments close at 5pm on 9 September 2013 Organisation (if applicable): Cairns and Far North Environment Centre Telephone: Address: PO Box 323N, Cairns North, QLD 4870 Section of TOR Describe the issue
LITHIUM, A STRATEGIC ELEMENT FOR ENERGY IN THE WORLD MARKET Robert Bruce Wallace∗ RESERVES AND RESOURCES STRUCTURE OF THE LITHIUM INDUSTRY VIII. MEXICO'S LITHIUM/POTASSIUM SALAR IX. I. INTRODUCTION This paper does not seek to be ground breaking originality, since numerous commentators and researchers, ranging from reporters, geologists, mining engineers, scientists of different fields and organizations, some economists, and official government institutions such as the United States Geologic Survey (USGS), have delved into the intricacies of the sources, production, demand, prices, competitive industrial structure, and even the geopolitics of lithium, its compounds, and its minerals for a good many years. What the paper does seek is to gather together different dispersed sources of information, both technical and economic, and present a coherent, critical general analysis. Furthermore, though there is unfortunately a lack of sufficient hard data regarding a pending development of what appears to be a huge lithium-potassium deposit in Mexico straddling the limits of Zacatecas and San Luis Potosí, I shall include an analysis of what has been divulged publicly via the internet, most of which is hopeful expectation.