Pii: s0896-6273(02)00623-2
Neuron, Vol. 33, 947–958, March 14, 2002, Copyright
2002 by Cell Press
Cellular Mechanisms of the Slow (⬍
1 Hz)
Oscillation in Thalamocortical Neurons In Vitro
Stuart W. Hughes,2 David W. Cope,2,3
relate to the slow rhythm is also characterized by re-
Kate L. Blethyn, and Vincenzo Crunelli1
peating up and down states, which often demonstrate
School of Biosciences
a stereotypical waveform (Steriade et al., 1993a, 1996;
Contreras and Steriade, 1995). In particular, the transi-
tion from up to down state is commonly initiated by a
Cardiff CF10 3US
pronounced inflection point, whereas the transition from
down to up state is marked by a low-threshold Ca2⫹
potential (LTCP) and accompanying high-frequency
burst of action potentials (see Figures 10, 11, and 12 in
Steriade et al., 1993a; Figures 8, 9, and 10 in Contreras
and Steriade, 1995; Figure 6 in Steriade et al., 1996;
Figure 9 in Contreras and Steriade, 1997b). The ensuing
The slow (⬍
1 Hz) rhythm is a defining feature of the
synaptic imposition of this burst of action potentials on
electroencephalogram during sleep. Since cortical cir-
cortical neurons has been strongly implicated in facilitat-
cuits can generate this rhythm in isolation, it is as-
ing synchronization of the slow rhythm, suggesting an
sumed that the accompanying slow oscillation in
important role for TC neurons in shaping its global attri-
thalamocortical (TC) neurons is largely a passive re-
butes (Contreras and Steriade, 1995; Contreras et al.,
flection of neocortical activity. Here we show, how-
ever, that by activating the metabotropic glutamate
The slow (⬍
1 Hz) oscillation in TC neurons is depen-
receptor (mGluR), mGluR1a, cortical inputs can recruit
dent on corticofugal input since it is abolished following
intricate cellular mechanisms that enable the genera-
decortication (Timofeev and Steriade, 1996). This has
tion of an intrinsic slow oscillation in TC neurons in
led to the belief that it is primarily a simple reflection of
vitro with identical properties to those observed in
rhythmic cortical activity (Steriade et al., 1993a). How-
vivo. These mechanisms rely on the "window" compo-
nent of the T-type Ca2ⴙ
current and a Ca2ⴙ
-activated,
ever, in TC neurons maintained in vitro, where corticofu-
nonselective cation current. These results suggest an
gal input is absent, we have recently described an intrin-
active role for the thalamus in shaping the slow (
sic slow oscillation possessing a similar appearance to
Hz) sleep rhythm.
that observed in vivo (see Figures 6 and 7 in Williams
et al., 1997). This oscillation is present in a subset
(⬍
15%) of cells and is generated by a bistable interac-
tion between the "window" (i.e., steady-state) compo-
nent of the T-type Ca2⫹
current, IT, ITwindow, and the leak
During deep sleep, the electroencephalogram (EEG) of
K⫹
current, ILeak (Williams et al., 1997) (see Supplemental
humans and animals is dominated by large amplitude,
Figure S1 at http://www.neuron.org/cgi/content/full/33/
slow waves that recur every 1–10 s (Steriade et al.,
6/947/DC1). Since this interaction only occurs if gLeak is
1993b, 1993c, 1993d, 2001; Achermann and Borbe´ly,
below a specific threshold, we were able to show that
1997; Amzica and Steriade, 1997; see also Simon et al.,
the artificial reduction of gLeak, using a dynamic clamp
2000). This so-called slow (⬍
1 Hz) rhythm is generated
system, brings about a slow oscillation in all TC neurons
in neocortical networks (Steriade et al., 1993c, 1993d;
(Hughes et al., 1999). As a major effect of corticofugal
Sanchez-Vives and McCormick, 2000) and organizes
input onto TC neurons is to reduce gLeak via the activation
other sleep rhythms, such as spindle and ␦
waves, into
of postsynaptic metabotropic glutamate receptors
coherent, repeating episodes (Steriade et al., 1993a,
(mGluRs) (McCormick and von Krosigk, 1992), we hy-
1993b, 1993d). At the cellular level, the slow rhythm is
pothesized that activation of this modulatory compo-
evident in all types of cortical neurons and is manifested
nent of cortical influence alone should initiate a slow
as recurring sequences of synaptic barrages that can
oscillation in these cells.
lead to epochs of sustained action potential firing, inter-
In this study, we demonstrate that in TC neurons of the
spersed with periods of disfacilitation or quiescence
cat dorsal lateral geniculate nucleus (LGN) maintained in
(Steriade et al., 1993c). This pattern of activity leads to
vitro, exogenous or synaptic activation of the postsyn-
prominent "up" and "down" states of the membrane
aptic mGluR associated with cortical input, mGluR1a
potential and is thought to be generated by a combina-
(Godwin et al., 1996), indeed leads to an intrinsic slow
tion of extensive interconnectivity in the neocortex and
(⬍
1 Hz) oscillation with identical properties to those
the intrinsic properties of cortical neurons (Amzica and
observed in vivo. As predicted, this mGluR1a-induced
Steriade, 1995; Sanchez-Vives and McCormick, 2000).
oscillation depends on an ITwindow-mediated bistability
In thalamocortical (TC) neurons, the intracellular cor-
(Williams et al., 1997; Hughes et al., 1999). In addition,
a Ca2⫹
-activated, nonselective cation current (ICAN) is es-
sential for stabilizing and enhancing the expression of
2 These authors contributed equally to the work.
3
the slow oscillation. These results suggest that TC neu-
Present address: MRC Anatomical Neuropharmacology Unit, De-
rons actively participate in sculpting the slow (⬍
1 Hz)
partment of Pharmacology, University of Oxford, Mansfield Road,
Oxford OX1 3TH, United Kingdom
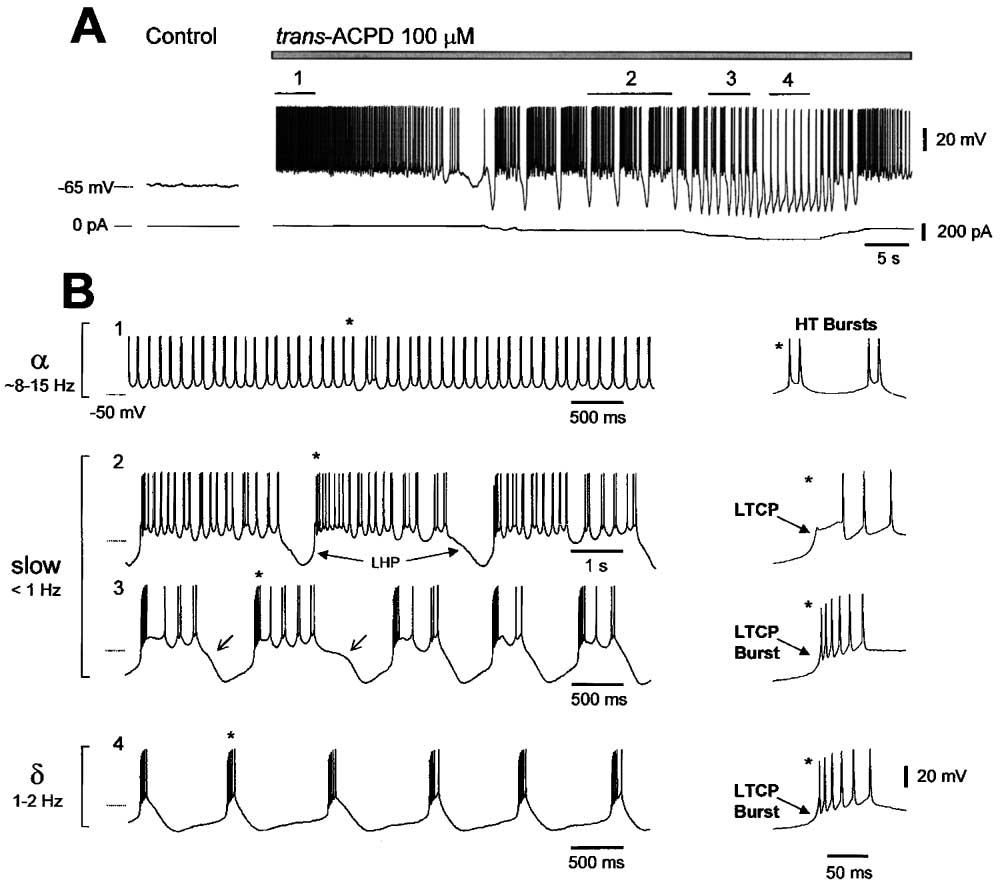
Figure 1. Instatement of a Slow Oscillation in TC Neurons Following the Application of trans-ACPD
(A) A TC neuron recorded in control conditions exhibiting a resting membrane potential of ⫺65 mV and a lack of spontaneous firing. Following
trans-ACPD (100 M) application, the neuron depolarizes and generates rhythmic spike doublets, i.e., high-threshold (HT) burst firing, at ⵑ10
Hz (see [B1]). Injection of hyperpolarizing d.c. current reveals a slow oscillation (see [B2 and B3]).
(B) The oscillation consists of recurring up and down states of the membrane potential (2 and 3). Each down state is initiated by a clear
inflection point ( in [3]) leading to a stereotypical large hyperpolarizing potential (LHP) that is terminated by a low-threshold Ca2⫹ potential
(LTCP). The LTCP is followed by a prolonged depolarization or up state consisting of HT bursting. The frequency of the oscillation increases
with hyperpolarizing d.c. current, with the oscillation eventually becoming a pure ␦ oscillation (4).
mick and Pape, 1990; Leresche et al., 1991). The pres-
ence of the slow oscillation was dependent on the con-
Application of trans-ACPD Leads to the
centration of trans-ACPD, with a lower concentration
Generation of a Slow Oscillation
(50 M) leading to a reduced prevalence (n ⫽ 4 of 20;
In control conditions, TC neurons exhibited a resting
20%) accompanied by a smaller depolarization (⌬V ⫽
membrane potential of ⫺66 ⫾ 1 mV and therefore did
11 ⫾ 3 mV), while a higher concentration (125 M) led
not exhibit spontaneous action potential firing (n ⫽ 83)
to an increased prevalence (n ⫽ 8 of 10; 80%) accompa-
(Figure 1A). However, application of the nonspecific
nied by a larger depolarization (⌬V ⫽
21 ⫾ 3 mV).
Group I/II mGluR agonist, trans-ACPD (100 M), depo-
The trans-ACPD-induced slow (⬍1 Hz) oscillation was
larized TC neurons (⌬V ⫽
17 ⫾ 1 mV; n ⫽ 83) and
characterized by prominent up and down states, which
typically caused them to exhibit either sustained tonic
were separated by 15–25 mV (Figures 1A and 1B). This
firing (n ⫽ 52) or repetitive high-threshold (HT) (⬎⫺55
led to a bimodal distribution of membrane potential with
mV) bursting at alpha (␣) (8–15 Hz) or theta () (3–7 Hz)
the two modes located between ⵑ⫺75 to ⫺65 mV and
frequencies (n ⫽ 15) (Figures 1A and 1B1). In addition,
ⵑ⫺55 to ⫺45 mV (see Supplemental Figure S2B at http://
the majority of neurons (n ⫽ 54 of 83; 65%) became
able to generate a slow (⬍1 Hz) membrane potential
down state was encompassed in a stereotypical large
oscillation (Figures 1A, 1B2, and 1B3). The frequency of
hyperpolarizing potential (LHP) (Figure 1B2) that was ini-
the slow oscillation increased with hyperpolarizing d.c.
tiated by a clear inflection point (see in Figure 1B3).
current (minimum frequency ⫽ 0.32 ⫾ 0.04 Hz; n ⫽ 54)
Following the peak of the LHP, the membrane potential
(Figures 1B2 and 1B3 and see Supplemental Figure S2A
exhibited a slow depolarization (Figure 1B3) due to the
activation of the mixed cation current, Ih (McCormick
DC1), with larger values leading to a continuous ␦ (1–2
and Pape, 1990; Soltesz et al., 1991), which eventually
Hz) oscillation (Figure 1B4) (Steriade et al., 1991; McCor-
gave rise to an LTCP (Crunelli et al., 1989) (see also
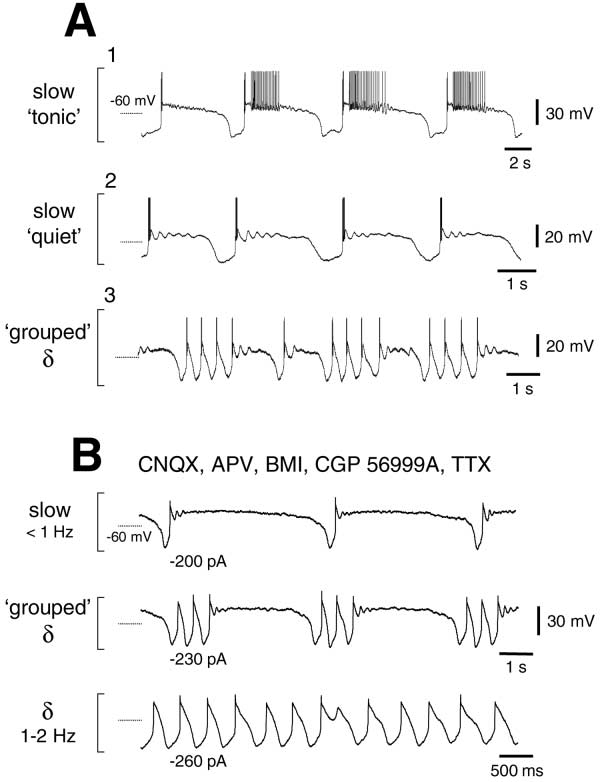
The Slow (⬍1 Hz) Oscillation in TC neurons
Figures 6B and 6C). This LTCP did not always support
a high-frequency burst of action potentials (Figure 1B2),
which may reflect an increased inactivation of T-type
Ca2⫹ channels due to an mGluR-induced dendritic depo-
larization (Zhan et al., 2000). The LTCP was followed by
a prolonged depolarization of variable duration, which
comprised the up state of the oscillation (Figures 1B2
and 1B3).
Different Manifestations of the Slow Oscillation
and Grouping of the ␦ Oscillation
The trans-ACPD-induced slow oscillation was apparent
as one of three main variations depending on the nature
of the up state, which either (1) gave rise to periods of
HT bursting at ␣ or frequencies (n ⫽ 7; 13%) (Figures
1B2 and 1B3 and see Supplemental Figure S3 at http://
www.neuron.org/cgi/content/full/33/6/947/DC1); (2)
consisted of a combination of periods of either sus-
tained tonic firing or quiescence (n ⫽ 14; 26%) (Figure
2A1); or (3) comprised quiescent periods only (n ⫽ 33;
61%) (Figure 2A2). In some neurons (n ⫽ 14), the slow
oscillation "grouped" periods of ␦ oscillations into short
recurring episodes. This was apparent as a replacement
of the LHP with a few cycles of rhythmic LTCPs at ␦
(1–2 Hz) frequencies (Figures 2A3 and 2B). This grouped
␦ activity occurred as a transition stage between a con-
tinuous ␦ oscillation and a "pure" slow oscillation (Figure
2B and see Supplemental Figure S3 at http://www.neuron.
Figure 2. Different Manifestations of the trans-ACPD-Induced Slow
org/cgi/content/full/33/6/947/DC1). Neither the slow os-
Oscillation and Its Intrinsic Nature
cillation nor its ability to group ␦ oscillations depends
(A) Traces from three different TC neurons show examples of (1) a
on non-NMDA, NMDA, GABAA, or GABAB receptors
slow oscillation that exhibits up states that consist mainly of periods
since they were unaffected by CNQX (10 M), APV (100
of tonic firing (slow "tonic") (cf. Figure 6A in Steriade et al., 1996);
M), BMI (30 M), and CGP 56999A (10 M) (n ⫽ 16
(2) a slow oscillation comprising isolated LTCP bursts followed by
of 16) (Figure 2B). In addition, the slow oscillation and
quiescent up states (slow "quiet") (cf. Figures 9A and 10B in Con-
grouping of ␦ oscillations were insensitive to tetrodotoxin
treras and Steriade, 1995); and (3) grouping of the ␦ oscillation by
the slow oscillation (cf Figures 10 and 12 in Steriade et al., 1993a
(TTX; 1 M) (n ⫽ 9 of 9) (Figure 2B), illustrating that they
and Figure 9 in Contreras and Steriade, 1997b). (Note: action poten-
are due to a direct postsynaptic effect of trans-ACPD.
tials have been truncated for clarity).
(B) Slow oscillation and grouping of the ␦ oscillation in a TC neuron
The Effect of trans-ACPD Is Fully Accounted
recorded in the presence of CNQX (10 M), APV (100 M), BMI (30
for by a Reduction of gLeak
M), CGP 56999A (10 M), and TTX (1 M). Traces acquired prior
In control conditions, the voltage response of TC neu-
to TTX application are shown in Supplemental Figure S3 at http://
www.neuron.org/cgi/content/full/33/6/947/DC1.
rons to small (10–50 pA) hyperpolarizing d.c. current
trans-ACPD was present in all experiments depicted in this figure).
pulses elicited from ⫺60 mV was monophasic, with cells
exhibiting an apparent input resistance of 192 ⫾ 15 M⍀
(n ⫽ 83) (Figures 3A1 and 3B1). Following the application
not observed in response to small current pulses, with
of 100 M trans-ACPD in neurons that exhibited the
cells continuing to exhibit monophasic charging, albeit
slow oscillation, this response was markedly altered,
with a 50% ⫾ 12% (n ⫽ 29) increase in apparent input
with small current pulses causing a stereotypical hyper-
resistance (Figures 3B1 and 3B2). However, a prominent
polarizing voltage waveform that was the same as the
ADP was unmasked in these neurons (Figure 3B2) (cf.
LHP evident during the slow oscillation (Figures 3A2 and
Turner and Salt, 2000), suggesting that different mecha-
3A3). In these neurons, the apparent input resistance in
nisms underlie the LHP and ADP. As expected from
the range ⫺60 to ⫺80 mV could be as high as 2.5 G⍀
previous studies (Williams et al., 1997; Hughes et al.,
(1.01 ⫾ 0.1 G⍀; n ⫽ 54), leading to an overall increase
1999), the apparent input resistance in control condi-
of 419% ⫾ 81% (n ⫽ 54). The current pulse-induced
tions was found to be a good predictor of whether or
LHP was always terminated by an LTCP that, in turn,
not TC neurons would exhibit an LHP-ADP sequence,
was always followed by a slowly decaying (up to 30
and thus a slow oscillation, following trans-ACPD appli-
s) afterdepolarization (ADP). This ADP was also clearly
cation, with nonoscillating cells displaying a significantly
apparent during the up state of the slow oscillation (Fig-
lower predrug value (113 ⫾ 19 M⍀; n ⫽ 29) than oscillat-
ure 3A3). Thus, the essential features of the slow oscilla-
ing cells (220 ⫾ 17 M⍀; n ⫽ 54) (p ⬍ 0.001). Accordingly,
tion were reproduced through the injection of hyperpo-
the likelihood of observing a slow oscillation following
larizing current pulses.
trans-ACPD application increased as a function of the
In neurons that did not exhibit the slow oscillation
apparent input resistance in control conditions. Neurons
following trans-ACPD application, an LHP response was
exhibiting values ⬍100 M⍀ never generated a slow oscil-
LHP and ADP can be explained by a simple reduction
in gLeak, the outcome of applying trans-ACPD and of
artificially reducing gLeak with a dynamic clamp system
(Hughes et al., 1999) was compared in the same TC
neuron (n ⫽ 5). By performing this procedure, it was
found that the effects of certain levels of artificial gLeak
reduction (g
4.0 ⫾ 0.7 nS) and trans-ACPD appli-
cation (125 M) were indistinguishable (Figure 3C). For
both scenarios, small hyperpolarizing current pulses
caused an identical LHP-ADP sequence (Figure 3C). In
addition, the effects of trans-ACPD were fully reversed
following an equivalent dynamic clamp-induced artificial
increase in g
Leak (gLeak
4.0 ⫾ 0.7 nS) (Figure 3C2).
Thus, the appearance of the LHP and ADP following
trans-ACPD application can be fully accounted for by a
reduction in gLeak.
The Slow Oscillation Arises through the Activation
of mGluR1a
The actions of trans-ACPD in bringing about the slow
(⬍1 Hz) oscillation were mimicked by the Group I mGluR
(i.e., mGluR1a and mGluR5) agonist, DHPG (50 M; n ⫽
6 of 9) (Figure 4A). Correspondingly, DHPG depolarized
(⌬V ⫽
11 ⫾ 2 mV; n ⫽ 9) TC neurons and caused an
increase in apparent input resistance (463% ⫾ 275%;
n ⫽ 9) (Figures 4A and 4B). In contrast, neither the
mGluR5 agonist CHPG (1 mM; n ⫽ 4), the Group II mGluR
agonists APDC (100–200 M; n ⫽ 5) and DCG-IV (10–100
M), nor the Group III mGluR agonist L-AP4 (100–200
M; n ⫽ 4) caused either a slow oscillation, a change
in membrane potential, or an increase in apparent input
resistance (p ⬎ 0.75) (Figure 4B).
To confirm that trans-ACPD brings about a slow oscil-
lation through the activation of mGluR1a, a number of
Figure 3. The Effects of trans-ACPD Are Fully Accounted for by a
mGluR subtype-specific antagonists were tested on the
Reduction in g
response of TC neurons to 100 M trans-ACPD. The
(A) In control conditions, the voltage response of a TC neuron, that
mGluR1a antagonists LY367385 (250 M; n ⫽ 10),
displayed the slow oscillation following trans-ACPD application, to
CPCCOEt (300 M; n ⫽ 4), and AIDA (250 M; n ⫽ 3)
a small hyperpolarizing current pulse (⫺40 pA) consists of a mono-
all led to a significant (p ⬍ 0.001) reversal of both the
phasic charging pattern (1). trans-ACPD (100 M) causes an in-
trans-ACPD-induced depolarization (96% ⫾ 2%, 72% ⫾
crease in apparent input resistance (2) and a modified charging
7%, and 65% ⫾ 12%, respectively) and increase in ap-
pattern comprising a stereotypical LHP. The LHP is followed by
parent input resistance (82% ⫾ 11%, 80% ⫾ 12%, and
an LTCP and afterdepolarization (ADP). The current pulse-induced
LHP-ADP sequence ([3], left trace) is equivalent to the activity evi-
55% ⫾ 30%, respectively) (Figures 4C and 4D). In addi-
dent during a cycle of the slow oscillation ([3], right trace).
tion, both LY367385 and CPCCOEt abolished the slow
(B) In a neuron that did not display the slow oscillation, trans-ACPD
oscillation in all cases (n ⫽ 8 of 8 and n ⫽ 3 of 3,
(100 M) causes an increase in apparent input resistance (1 and 2)
respectively), whereas AIDA eliminated the slow oscilla-
and unmasks a prominent ADP (2), but does not lead to the appear-
tion in two out of three cases (Figures 4C and 4D). In
ance of an LHP (2).
contrast, neither the mGluR5 antagonist MPEP (30 M;
(C) A neuron exhibiting a conventional charging pattern in response
to small hyperpolarizing current steps (⫺20 and ⫺40 pA) ([1], left
n ⫽ 4) (Figures 4C and 4D), the Group II mGluR antago-
trace). Following an artificial reduction in g
Leak (artificial gLeak
nist EGLU (250 M; n ⫽ 4), nor the Group II/III mGluR
using a dynamic clamp system, the neuron displays a characteristic
antagonist CPPG (n ⫽ 3) inhibited either the slow oscilla-
LHP-ADP sequence in response to a 20 pA current step ([1], right
tion or the trans-ACPD-induced depolarization and in-
trace). In the same neuron, an identical LHP-ADP sequence is appar-
crease in apparent input resistance (p ⬎ 0.75) (Figure 4D).
ent following trans-ACPD application ([2], left trace). A subsequent
artificial increase in gLeak ([2], right trace) causes the neuron to revert
to activity identical to that observed in control conditions (1, left
Activation of mGluR1a through the Stimulation
trace). TTX (1 M) was also present in the recording medium. (Note:
of Corticothalamic Fibers Also Causes
action potentials in [A] and [B] have been truncated for clarity).
a Slow Oscillation
In order to test whether the physiological release of
lation, while neurons exhibiting values in the ranges 100–
glutamate can cause the appearance of the slow (⬍1
150 M⍀ and 151–200 M⍀ generated a slow oscillation
Hz) oscillation, the response of TC neurons to tetanic
in 42% and 75% of cases, respectively, and neurons
stimulation of corticothalamic (CT) fibers was examined.
exhibiting values ⬎200 M⍀ did so in 92% of cases.
In a substantial number of cells (n ⫽ 9 of 16; 56%)
In order to demonstrate that the appearance of the
that showed no rhythmic activity in control conditions,
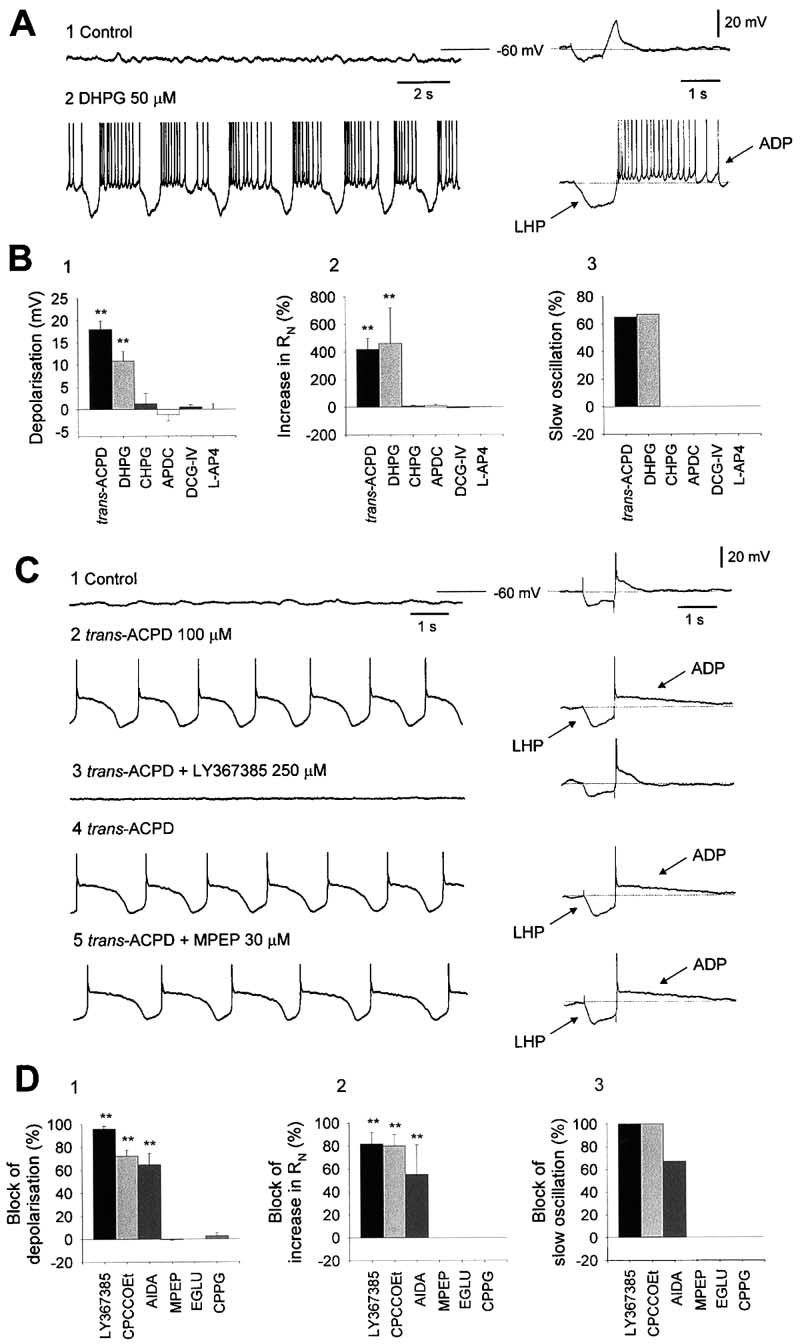
The Slow (⬍1 Hz) Oscillation in TC neurons
Figure 4. The trans-ACPD-Induced Slow Os-
cillation Arises through the Activation of
mGluR1a
(A) Application of DHPG (50 M) to a TC neu-
ron leads to the generation of a slow oscilla-
tion and a characteristic LHP-ADP sequence
in response to a small hyperpolarizing current
pulse (40 pA).
(B) Summary of the response of TC neurons
to the application of various mGluR agonists
(R ⫽
apparent input resistance; % of cells).
(C) In control conditions, a TC neuron shows
a lack of oscillatory activity (left trace) and no
LHP-ADP sequence in response to a small
current pulse (50 pA) (right trace) (1). trans-
ACPD (100 M) causes a slow oscillation (left
trace) and the appearance of an LHP-ADP
response (right trace) (2). Both the slow oscil-
lation and the LHP-ADP response are revers-
ibly abolished by the mGluR1a antago-
nist, LY367385 (250 M) (3 and 4), but are
unaffected the mGluR5 antagonist, MPEP
(30 M) (5).
(D) Summary of the effect of various mGluR
antagonists on the response of TC neurons
to 100 M trans-ACPD. (R ⫽
resistance; % of cells; ** p ⬍ 0.001)
following the offset of the stimulation epoch a damped
of CT fibers when TC neurons were held at an initial
oscillation was generated that consisted of rhythmic
membrane potential negative to ⫺70 mV caused a long-
LTCP-mediated bursts that recurred with an initial
lasting EPSP (amplitude ⫽ 4.5 ⫾ 0.4 mV; n ⫽ 6) (Figure
frequency ⬍1 Hz (0.91 ⫾ 0.07 Hz). In addition, these
5A4) (cf. McCormick and von Krosigk, 1992; Turner and
LTCP-mediated bursts were often followed by a pro-
Salt, 1998, 2000; von Krosigk et al., 1999). Thus, all the
longed up state that was terminated by a characteristic
actions of trans-ACPD could be mimicked by the electri-
inflection point (Figure 5A2). The unmasking of the slow
cal stimulation of CT fibers.
oscillation by physiological stimuli was dependent on
The amplitude of the slow EPSP was enhanced by
membrane potential, requiring the initial resting value
blockers of GABA and ionotropic glutamate receptors
to be in the region of ⫺65 to ⫺60 mV. In contrast, stimula-
(i.e., CNQX, APV, BMI, and CGP 56999A) (7.8 ⫾ 1.0 mV;
tion when the initial value was ⬎⫺60 mV typically led
n ⫽ 10; p ⬍ 0.01) (see Supplemental Figure S4A at http://
to either tonic firing (cf. McCormick and von Krosigk,
1992) or HT bursting (Figure 5A1), while stimulation when
Turner and Salt, 1998; von Krosigk et al., 1999). Accord-
the initial value was between ⫺70 and ⫺65 mV usually
ingly, the prevalence of the stimulus-induced slow oscil-
caused a damped ␦ oscillation (Figure 5A3). Stimulation
lation was increased from 56% to 80% (see Supplemen-
Figure 5. Stimulation of Corticothalamic Fibers Also Leads to the Generation of an mGluR1a-Dependent Slow Oscillation
(A) Effects of CT stimulation on TC neurons in the absence of trans-ACPD. Tetanic stimulation when the membrane potential is ⫺57 mV gives
rise to a mixed period of HT bursting and tonic firing (1). Stimulation when the membrane potential is more hyperpolarized brings about a
slow oscillation (⫺60 to ⫺65 mV) (2) or a ␦ oscillation (⫺65 to ⫺70 mV) (3). Note the prolonged depolarized phase following the LTCPs in (2)
and the ensuing characteristic inflection point (). When the membrane potential is negative to ⫺70 mV, CT stimulation reveals an underlying
slow EPSP (4).
(B) TC neuron recorded in the presence of CNQX (10 M), APV (100 M), BMI (30 M), CGP 56999A (10 M), EGLU (250 M), CPPG (50 M),
and MPEP (30 M) showing HT bursting (1), a slow oscillation (2), a ␦ oscillation (3), and an underlying slow EPSP (4) in response to CT
stimulation. LY367385 (250 M) abolishes all types of poststimulus oscillatory activity (5–7) and blocks the underlying slow EPSP (8) (see also
Supplemental Figure S4 at http://www.neuron.org/cgi/content/full/33/6/947/DC1).
tal Figure S4A at http://www.neuron.org/cgi/content/
bistability) (n ⫽ 19 of 19) (Figure 6A). This bistability is
full/33/6/947/DC1). However, subsequent introduction
caused by an interaction between ITwindow and ILeak that
of blockers of muscarinic, H
occurs when g
1, ␣1, and 5HT1/5HT2 recep-
Leak falls below a well-defined threshold
tors (with scopolamine, pyrilamine, prazosin, and meth-
(Williams et al., 1997; To´th et al., 1998; Hughes et al.,
ysergide, all at 3
1999) (see Supplemental Figure S1 at http://www.
M) did not affect either the amplitude
of the slow EPSP (6.9
neuron.org/cgi/content/full/33/6/947/DC1). The two sta-
⫾ 0.6 mV; n ⫽ 4; p ⫽ 0.51) or the
ble up and down states comprising the bistability could
prevalence of the stimulus-induced slow oscillation (75%)
be "switched" between by applying small current steps
(see Supplemental Figure S4A at http://www.neuron.
(cf. Williams et al., 1997; Hughes et al., 1999). As with
org/cgi/content/full/33/6/947/DC1). Similarly, no change
the slow oscillation, the transition from the up to down
in the properties or prevalence of the stimulus-induced
stable state was initiated by a marked inflection point,
slow oscillation was observed following the subsequent
while the shift from the down to up stable state was
addition of either a combination of EGLU (250 M) and
characterized by an LTCP and transient ADP (Figure
CPPG (50 M) (EPSP amplitude ⫽ 7.6 ⫾ 0.5 mV; n ⫽ 4;
6A2). A comparison of the ADP generated in normal con-
p ⫽ 0.97) (prevalence of slow oscillation ⫽ 75%), or
ditions with that exhibited following a block of Ih revealed
MPEP (50 M) (EPSP amplitude ⫽ 6.8 ⫾ 0.3 mV; n ⫽ 4;
no differences in either amplitude (14.3 ⫾ 1.4 mV versus
p ⫽ 0.41) (prevalence of slow oscillation ⫽ 67%) (see
14.8 ⫾ 1.3 mV; p ⫽ 0.78) or duration (3.8 ⫾ 0.5 s versus
Supplemental Figure S4A at http://www.neuron.org/cgi/
3.9 ⫾ 0.6 s; p ⫽ 0.86) (n ⫽ 10 neurons) (Figure 6A3).
content/full/33/6/947/DC1). However, LY367385 (250
Bistability and the LTCP were unaffected by TTX (1
M; n ⫽ 6) abolished both the slow EPSP (p ⬍ 0.001)
M; n ⫽ 6) (Figure 6B) or by either of the K⫹ channel
and the stimulus-induced slow oscillation in all cases (Fig-
blockers, Ba2⫹ (1 mM; n ⫽ 5) or 4-AP (100–250 M; n ⫽
ure 5B and see Supplemental Figures S4A and S4B at
3) (data not shown). The high-voltage activated (HVA)
Ca2⫹ channel blockers Cd2⫹ (250–400 M; n ⫽ 3), nifedi-
pine (1–10 M; n ⫽ 3), -conotoxin-MVIIC (3 M; n ⫽
6), or -conotoxin-GVIA (3 M; n ⫽ 3) also failed to
Bistability Brought About by the Interaction of
affect either bistability or the LTCP (data not shown).
ITwindow and ILeak Underlies the mGluR1a-Induced
However, both bistability and the LTCP were selectively
abolished by Ni2⫹ (350–500 M; n ⫽ 4) (Figure 6B), con-
As expected from previous studies (Williams et al.,
firming that they are dependent on IT and, in particular,
1997), the Ih blocker ZD 7288 (50–300 M) abolished the
that bistability relies on ITwindow as opposed to other mech-
slow "sag" potential apparent during the LHP, leading
anisms (Chuang et al., 2000). These points were rein-
to an uncoupling of the up and down states of the slow
forced by observing that subsequent introduction of an
oscillation and the appearance of two distinct, stable
artificial I
124 ⫾ 36 nS; n ⫽ 5) using a dynamic
resting membrane potentials (i.e., membrane potential
clamp system reinstated bistability and the LTCP (Fig-
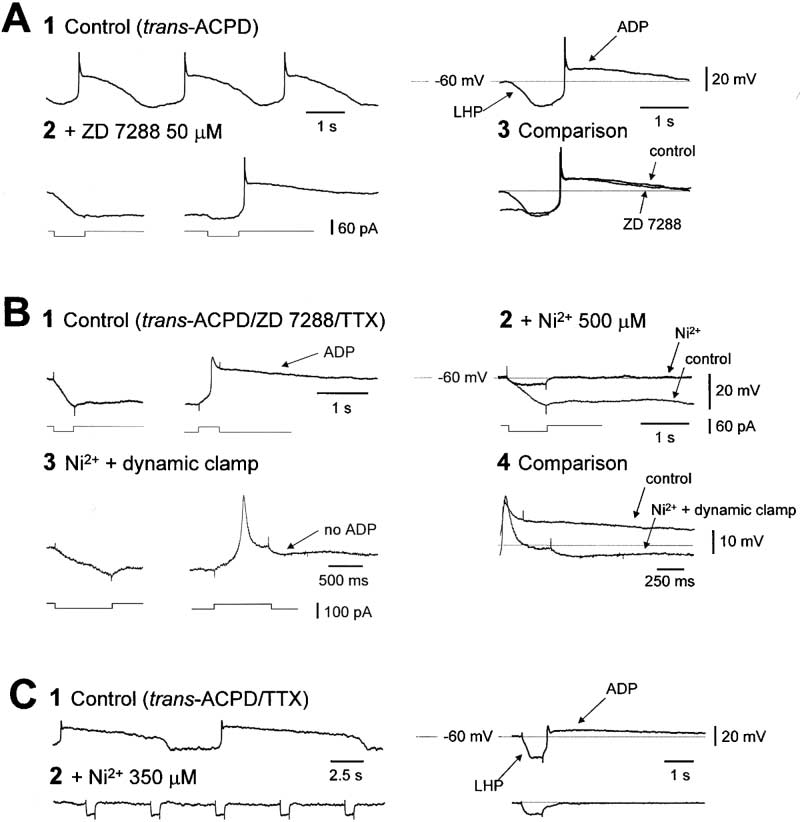
The Slow (⬍1 Hz) Oscillation in TC neurons
Figure 6. A Bistable Interaction between ITwindow
and ILeak Underlies the mGluR1a-Induced
Slow Oscillation
(A) In the presence of trans-ACPD, this neu-
ron exhibits a typical slow oscillation ([1], left
trace) as well as an LHP-ADP sequence in
response to a hyperpolarizing current pulse
(20 pA, 800 ms) ([1], right trace). The Ih blocker,
ZD 7288, uncouples the up and down states
of the slow oscillation, leading to two stable
membrane potentials (i.e., membrane poten-
tial bistability) at ⫺60 and ⫺78 mV that can be
"switched" between by appropriate current
steps (2). The ADPs generated before and
after application of ZD 7288 are identical (3).
(B) The LTCP and membrane potential bista-
bility recorded in a TC neuron in the presence
of trans-ACPD, ZD 7288, and TTX (1) are abol-
ished by Ni2⫹ (500 M) (2), but they are rein-
stated following the introduction of artificial
I
70 nS) using a dynamic clamp system
(3). However, artificial IT is unable to recreate
the ADP (4).
(C) A slow oscillation recorded in the pres-
ence of trans-ACPD and TTX ([1], left trace)
and the LHP-ADP response of the neuron to
a hyperpolarizing current pulse (50 pA, 1 s)
([1], right trace) are eliminated by Ni2⫹ (350
M) (2). (Note: action potentials in [A] have
been truncated for clarity).
ures 6B3 and 6B4) (see Hughes et al., 1999). The funda-
M; n ⫽ 6), -conotoxin-MVIIC (3 M; n ⫽ 6), or
mental reliance of the slow oscillation on ITwindow was
-conotoxin-GVIA (3 M; n ⫽ 3) (p ⬎ 0.5) (data not
conclusively illustrated by noting its abolition by Ni2⫹
shown), illustrating that it does not involve TTX-sensitive
(350–500 M; n ⫽ 5) (Figure 6C), but not by Cd2⫹ (250–
Na⫹ channels and is not dependent on HVA Ca2⫹ chan-
400 M; n ⫽ 4) (see Supplemental Figure S5A at http://
nels. In addition, Ba2⫹ (1 mM; n ⫽ 5) and 4-AP (100–250
M; n ⫽ 3) either did not affect the ADP or led to its
dipine (1–10 M; n ⫽ 4), Ba2⫹ (1 mM; n ⫽ 4), or 4-AP
enhancement due to an increase in apparent input resis-
(100–250 M; n ⫽ 2) (data not shown).
tance (data not shown), thus ruling out the possible
involvement of a Ca2⫹-inactivated K⫹ current (Tokimasa,
1985).
A Ca2ⴙ-Activated, Nonselective Cation Current
Replacement of NaCl with choline chloride failed to
Underlies the ADP
affect the ADP (n ⫽ 5) (data not shown). However, substi-
In addition to blocking membrane potential bistability,
tution of the majority (134 mM) of Na⫹ with NMDG⫹
the slow oscillation, and the LTCP, Ni2⫹ always abol-
reversibly inhibited the ADP (n ⫽ 7) (Figure 7B), sug-
ished the ADP (n ⫽ 9 of 9) (Figures 6B2 and 6C2). Further-
gesting that it is generated by a Ca2⫹-activated, nonse-
more, while artificial IT was able to restore bistability and
lective cation current, ICAN (Partridge and Swandulla,
the LTCP following their block by Ni2⫹, it was unable to
1988), as is the case for similar behavior in other thalamic
recreate the ADP (Figures 6B3 and 6B4). Since artificial
neurons (Bal and McCormick, 1993; Zhu et al., 1999). In
IT fully replicates the biophysical properties of endoge-
order to test this hypothesis, a hybrid current/voltage
nous IT (Hughes et al., 1999), except that it is not carried
clamp protocol was employed. Switching the amplifier
by Ca2⫹, it appeared that the ADP must involve a Ca2⫹-
from current to voltage clamp at the peak of the ADP
dependent conductance. This hypothesis was well sup-
revealed a small, slowly decaying inward current (Figure
ported by previous investigations in rat TC neurons
7C2). By measuring the difference in current between
(Turner and Salt, 2000) and by the observation in this
the peak of the ADP and at steady state when the ADP
study that the inclusion of either BAPTA (100 mM; n ⫽
has decayed, it was found that the current responsible
3) or EGTA (50–100 mM; n ⫽ 5) in the recording electrode
for the ADP (1) exhibited an amplitude (⫺21.7 ⫾ 6.4 pA
abolished the ADP without any loss in apparent input
at ⫺60 mV; n ⫽ 5) that was linearly related to membrane
resistance (Figure 7A).
potential (Figure 7C3), (2) showed an extrapolated rever-
The ADP was unchanged by TTX (1 M; n ⫽ 21) (see
sal potential of 8.7 ⫾ 1.1 mV (n ⫽ 5) (Figure 7C3), and
Supplemental Figure S5B at http://www.neuron.org/cgi/
(3) displayed a time-course that decayed monoexpo-
content/full/33/6/947/DC1), Cd2⫹ (250–400 M; n ⫽ 10)
nentially in a voltage-independent manner, with
(see Supplemental Figure S5A at http://www.neuron.
1.44 ⫾ 0.3 s (n ⫽ 5) (Figure 7C2). These measurements
were unaffected by TTX (1 M) and Ba2⫹ (1–2 mM) (am-
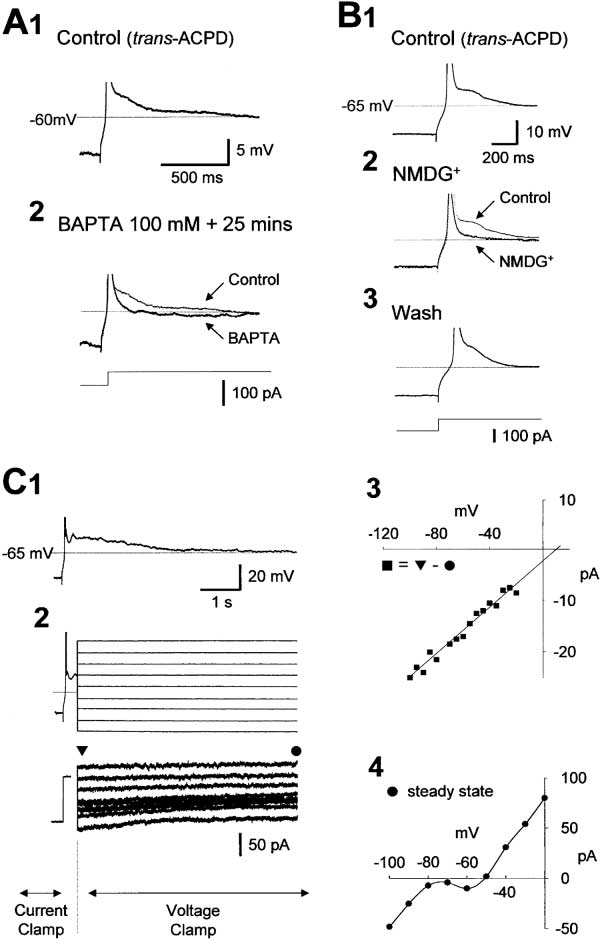
Simulations of the Slow Oscillation
The significance of ICAN in the slow (⬍1 Hz) oscillation
was assessed using a biophysical model of a TC neuron
(Williams et al., 1997). In the absence of I
CAN (gCAN
0 nS) (Figure 8A), the model exhibited conventional ␦
oscillations that showed a characteristic increase in fre-
quency over a wide range of injected d.c. current values
(⫺50 to ⫺10 pA). However, for larger values of injected
d.c. current, this region was followed by a rapid decline
in frequency such that the minimum frequency of the
oscillation was achieved at the most positive level of
injected d.c. current for which an oscillation existed (Fig-
ure 8A2). The voltage waveform of the oscillation at fre-
quencies below 1 Hz was similar to that observed experi-
mentally (Figure 8A1). However, the range of currents for
which a slow oscillation existed was extremely narrow
(⬍1.5 pA) ("slow" in Figure 8A
2). Thus, with gCAN
the model is unable to support the stable generation of
a slow oscillation and also does not exhibit grouped ␦
oscillations.
The model was next examined in the presence of ICAN
(Figure 8B). Under these conditions, although oscillatory
frequency showed an initial increase followed by a sharp
decrease in response to increasing injected d.c. current,
the sharp decrease was followed by a region where
oscillation frequency gradually decreased from 0.24 to
0.06 Hz over a relatively large range of injected d.c.
currents (75 pA, i.e., ⫺85 to ⫺10 pA, for g
("slow" in Figure 8B2). The mean rate of decrease of
frequency with respect to current for this region (0.0024
Hz pA⫺1) was similar to that observed in experiments
(e.g., 0.0023 Hz pA⫺1 or from 0.41 to 0.2 Hz over 90 pA
for the example shown in Figure 8C). Thus, ICAN stabilizes
the slow oscillation over a wide range of conditions. ICAN
Figure 7. The ADP Is Generated by a Ca2⫹-Activated, Nonselective
achieves this effect by transforming what would nor-
Cation Current, ICAN
mally be a ␦ oscillation (see region labeled "␦" in Figure
(A) Releasing a TC neuron from hyperpolarization immediately after
8A2) into a slow oscillation by temporarily depolarizing
impalement leads to the generation of an LTCP followed by an ADP
the cell following an LTCP into or beyond the region
(1). The ADP is abolished following infusion of the cell with BAPTA
(100 mM) for 25 min (2).
(labeled "slow" in Figure 8A2) where it can exhibit a slow
(B) The ADP in another TC neuron (1) is reversibly blocked by substi-
oscillation in the absence of ICAN (i.e., top trace in Figure
tuting 134 mM Na⫹ with NMDG⫹ (2 and 3).
8A1). The waveforms of the slow oscillation generated
(C) Typical example of the ADP in a TC neuron (1). Switching the
0 nS were very similar to those observed
recording amplifier from current to voltage clamp at the peak of the
experimentally (Figure 8C1). Furthermore, as a transition
ADP reveals a slowly decaying inward current (2). The "difference-
stage between a continuous ␦ oscillation (Figure 8B1,
current" (䊏) measured between the peak of the ADP (䉲) and at
steady state (䊉) (i.e., the current underlying the ADP) possesses an
bottom trace) and a "pure" slow oscillation (Figure 8B1,
amplitude that is linearly related to membrane potential and exhibits
top trace), the model exhibited grouped ␦ activity (Figure
an extrapolated reversal potential of ⫹10 mV (3). The steady-state
8B1, middle trace). The grouped ␦ activity occurs when
I-V relationship exhibits a pronounced "S" shape (4), which is due
the effect of ICAN following a single LTCP is insufficient
to ITwindow and indicates an underlying bistable system (see Supple-
to force a prolonged depolarization (i.e., produce a shift
mental Figure S1 at http://www.neuron.org/cgi/content/full/33/6/
into or beyond the slow region). However, since a sec-
947/DC1). (Note: 50 M ZD 7288 was present in all experiments
depicted in this figure. Action potentials have been truncated for
ond LTCP occurs before ICAN has significantly decayed,
the conductance of ICAN accumulates and becomes large
enough to facilitate a prolonged depolarization (Figure
plitude at
⫺60 mV ⫽ ⫺24.3 ⫾ 10.5 pA; p ⫽ 0.5; extrapo-
1, middle trace).
lated reversal potential ⫽ 11.7 ⫾ 4.7 mV; p ⫽ 0.8;
1.14 ⫾ 0.16 s; p ⫽ 0.5) (n ⫽ 4) and are consistent with
Extracellular Recordings Demonstrate the Normal
the properties of ICAN (see Zhu et al., 1999). Interestingly,
Occurrence of the Slow Oscillation
the current measured at steady state exhibited a pro-
Finally, in order to show that TC neurons normally exhibit
nounced S-shape (Figure 7C4), which is due to the pres-
slow oscillations in the absence of intracellular manipu-
ence of ITwindow and is indicative of a bistable system (see
lation, extracellular single unit recordings were per-
Figure 1 in Hughes et al., 1999; and Supplemental Fig-
formed in the presence of various concentrations of
ure S1 at http://www.neuron.org/cgi/content/full/33/6/
trans-ACPD. These recordings revealed that at concen-
trations of trans-ACPD between ⵑ50 and 100 M, TC
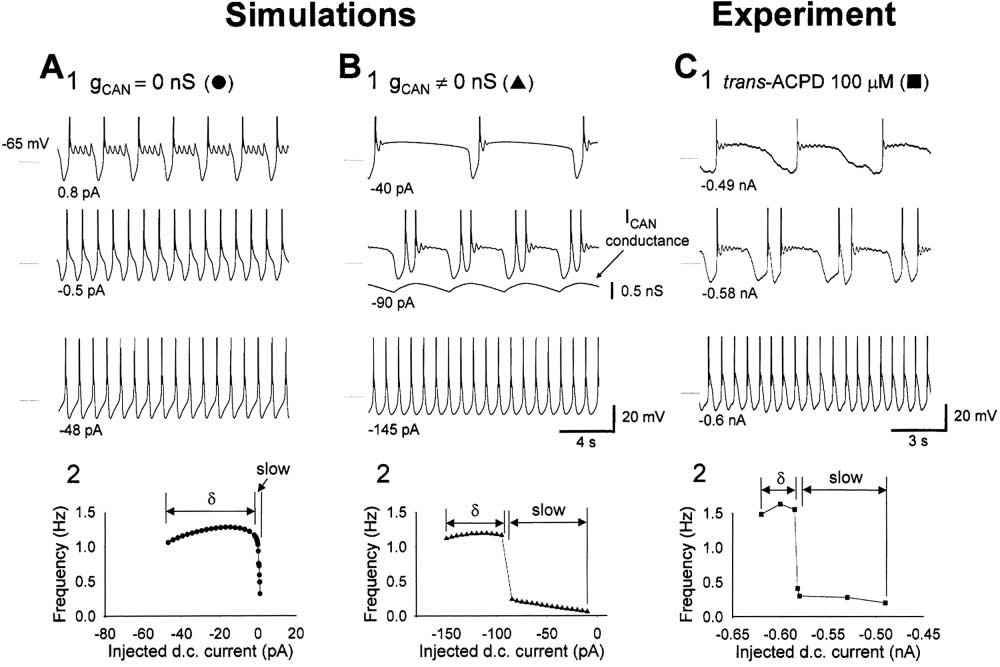
The Slow (⬍1 Hz) Oscillation in TC neurons
Figure 8. The Slow Oscillation in a Simplified Model of a TC Neuron
(A) In the absence of I
CAN (i.e., gCAN
0 nS), a model TC neuron with gLeak
1.5 nS exhibits a slow oscillation (1), but only for a very narrow
range of d.c. current inputs ("slow" in [2]).
(B) With g
10 nS, the model exhibits a slow oscillation over a much greater range of d.c. current inputs (1 and 2). Furthermore, the model
is able to exhibit grouped ␦ oscillations as a transition stage between a continuous ␦ oscillation and a pure slow oscillation. The grouped ␦
activity occurs while the conductance of ICAN is accumulating to a point where it is able to facilitate the generation of a prolonged up state.
(C) Experimental traces obtained in the presence of trans-ACPD (1) show almost identical properties to the simulations depicted in (B1). Also,
the current versus frequency plot for the experimental scenario (2) possesses a similar form to that shown in (B2).
neurons naturally demonstrate slow oscillations with
depends on a bistable interaction between ITwindow and
identical properties to those observed with intracellular
ILeak. The presence of this interaction in TC neurons can
recordings (see Supplemental Data and Supplemental
be easily detected, since a pharmacological block of Ih
Figure S6 at http://www.neuron.org/cgi/content/full/33/
instigates full membrane potential bistability (i.e., two
distinct stable up and down states) in these cells. Thus,
based on the following evidence, we conclude that the
slow oscillation portrayed here also results from an ITwindow-
ILeak bistable interaction, which is brought about solely
The main finding of this study is that exogenous or syn-
by an mGluR1a-induced reduction in gLeak, as set out in
aptic activation of mGluR1a causes an intrinsic slow
our initial hypothesis. First, the main reported effect of
(⬍1 Hz) oscillation in TC neurons. This slow oscillation
mGluR activation in TC neurons is to reduce gLeak
depends on a bistable interaction between ITwindow and a
(McCormick and von Krosigk, 1992; Turner and Salt,
reduced ILeak and is enhanced and stabilized by ICAN. This
2000), and in this study, all the effects of trans-ACPD
study therefore (1) shows that the slow (⬍1 Hz) sleep
on the properties of TC neurons relevant to the slow
rhythm has an intrinsic counterpart in individual neurons,
oscillation could be mimicked by a simple artificial re-
(2) describes a direct role for ITwindow in an important physi-
duction in gLeak. Second, following the application of ZD
ological activity of central neurons, (3) exposes both the
7288, cells that exhibit the slow oscillation following
presence and function of ICAN in TC neurons, and (4)
mGluR1a activation display membrane potential bista-
implies a role for mGluR1a in the generation of slow
bility. Third, this membrane potential bistability is
blocked by Ni2⫹ and can be subsequently reinstated by
artificial IT, but it is unaffected by other Ca2⫹ channel
Biophysical Mechanism Underlying the
blockers. Fourth, the slow oscillation is also selectively
mGluR1a-Induced Slow Oscillation
abolished by Ni2⫹. The most critical component in the
The slow (⬍1 Hz) oscillation described in this study
generation of the slow oscillation in TC neurons is there-
following mGluR1a activation possesses properties al-
most identical to those observed in previous studies in
The likelihood that TC neurons exhibit the slow oscilla-
a small sample of neurons with an unusually low g
tion following mGluR1a activation was dependent on
control conditions (Williams et al., 1997) or in all TC
their apparent input resistance in control conditions,
neurons following an artificial reduction in gLeak (Hughes
with larger values leading to an increased prevalence.
et al., 1999). In these neurons, slow oscillatory activity
This finding is consistent with the necessity for gLeak
to fall below a well-defined threshold before the slow
Comparison of the Slow Oscillation Observed in
oscillation can occur (To´th et al., 1998; Hughes et al.,
TC Neurons In Vivo and In Vitro
1999) and may explain why some previous investiga-
The properties of the slow (⬍1 Hz) oscillation described
tions into the effects of trans-ACPD on TC neurons have
in this study are entirely consistent with those observed
not observed activities similar to those described here
in vivo (Steriade et al., 1993a, 1996; Contreras and Steri-
(McCormick and von Krosigk, 1992; Lee and McCor-
ade, 1995, 1997a, 1997b), in that (1) the frequency ranges
of the slow oscillation in the two conditions are virtually
mick, 1997; Tennigkeit et al., 1999; Gutierrez et al., 2001;
identical; (2) in both cases, the slow oscillation is charac-
Zhan et al., 2000), while others have (Turner and Salt,
terized by prominent up and down states separated by
ⵑ20 mV, with the up state occurring around ⫺60 mV;
(3) in both scenarios, the transition from the up to down
state is commonly characterized by a marked inflection
The ADP and the Role of ICAN in the Slow Oscillation
point, while the transition from the down to up state is
Regardless of whether or not TC neurons displayed the
punctuated by an LTCP; (4) in vivo, the down state is
slow oscillation following mGluR1a activation, a pro-
associated with the highest value of apparent input re-
nounced ADP was always unmasked. Since an identical
sistance in the slow oscillation cycle (Contreras et al.,
ADP could be brought about by an artificial reduction
1996), whereas in vitro, the down state is necessarily
in gLeak, its appearance can be fully ascribed to an
related to a large apparent input resistance; (5) in similar-
mGluR1a-induced reduction in gLeak. Five main experi-
ity to that observed in vivo (Steriade et al., 1996; Con-
mental observations support our conclusion that the
treras and Steriade, 1997a), the depolarizing phase of
ADP is generated by ICAN (Partridge and Swandulla,
the slow oscillation shown in this study could consist
1988). First, artificial IT does not promote an ADP. Sec-
of periods of fast oscillations (i.e., tonic firing and HT
ond, the ADP is vulnerable to intracellular Ca2⫹ chelation.
bursting); (6) the overall proportion of cells in vitro exhib-
Third, the ADP is unaffected by ZD 7288. Fourth, the ADP
iting a slow oscillation that gave rise to fast oscillations
is dependent on extracellular Na⫹. Fifth, the biophysical
during the depolarizing phase (21 of 83; 25%) is similar
properties of the transient inward current generated fol-
to that observed in vivo (20%) (Steriade et al., 1996); (7)
lowing an LTCP are consistent with ICAN, as described
the slow oscillation in vitro groups ␦ oscillations in an
in other studies (Zhu et al., 1999). This current has proba-
indistinguishable manner to that observed in vivo; (8) as
bly not been appreciated in previous studies of TC neu-
is the case in vivo, the slow oscillation in vitro exists at
rons because of its masking by a larger ILeak in control
a level of depolarization between that of a continuous
␦ oscillation and sustained firing, and grouped ␦ activity
Computer simulations confirmed that ICAN is crucial to
occurs at a more hyperpolarized level than a pure slow
the generation of the slow oscillation. In the absence of
oscillation (Contreras and Steriade, 1997b); and (9) in
vivo, the slow oscillation is dependent on an intact cor-
CAN, the model was unable to provide a full explanation
for the slow oscillation observed experimentally, since
tex (Timofeev and Steriade, 1996), whereas in vitro, the
oscillations at frequencies
slow oscillation is unmasked when cortical input is mim-
⬍1 Hz could only be ob-
icked through either the exogenous or endogenous acti-
served for a very narrow range of injected d.c. currents.
vation of mGluR1a.
The presence of ICAN modifies this scenario by trans-
forming what would usually be a conventional ␦ oscilla-
A Role for mGluR1a in the Regulation
tion into a slow oscillation by transiently depolarizing
of Slow Sleep Rhythms
the cell following an LTCP into or beyond the narrow
It had previously been largely believed that the slow
region where a slow oscillation can exist in the absence
oscillation occurring in TC neurons in vivo was essen-
of ICAN. Thus, while ITwindow is responsible for the basic up
tially a passive reflection of cortical activity (Steriade et
and down states of the slow oscillation, the augmen-
al., 1993a). However, this study clearly shows that corti-
tation and prolongation of the up state by ICAN is crucial
cal input to TC neurons can induce slow rhythmicity
in order to stabilize and enhance the expression of the
by recruiting intrinsic mechanisms. That corticothalamic
oscillation over a wide range of conditions. In addition,
mGluRs are substantially activated during sleep is well
due to the ability of ICAN to "build up" over a number of
supported by numerous studies showing that in this
LTCP events, its inclusion in the model is also able to
behavioral state, cortical neurons continue to generate
fully explain the experimental observation of grouped ␦
sustained periods of action potential firing (Steriade et
oscillations (Steriade et al., 1993a).
al., 2001). Indeed, although the rhythmic recurrence of
A similar ADP has recently been described in LGN TC
hyperpolarizing episodes (i.e., the down states of the
neurons of the ferret (Lu¨thi and McCormick, 1998). In
slow oscillation; see Introduction) in cortical neurons will
contrast with our results, this ADP is thought to be gen-
lessen the overall output of the cortex to the thalamus in
erated by a Ca2⫹-mediated upregulation of Ih and is be-
comparison to wakefulness, the up state of the slow
lieved to be, at least in part, responsible for the waxing
oscillation in cortical neurons supports action potential
and waning of thalamic spindle oscillations (Bal and
firing rates that are comparable to those observed dur-
McCormick, 1996). However, the lack of effect of the
ing the wake state (Steriade et al., 2001).
specific Ih-blocker, ZD 7288, on the ADP observed in this
study suggests that Ih is not involved in its generation.
Rather, the role of Ih in the slow oscillation is to dictate
Slice Preparation and Maintenance
the duration of the stereotypical down state, or LHP,
All procedures involving experimental animals were carried out in
and facilitate the transition from the down to up state.
accordance with the UK Animals (Scientific Procedure) Act, 1986
The Slow (⬍1 Hz) Oscillation in TC neurons
and local ethics committee guidelines. All efforts were made to
were similar to those observed experimentally in the presence of
minimize animal suffering and the number of animals used. Young
trans-ACPD. In order to describe ICAN, we adapted a kinetic scheme
adult cats (1–1.5 kg) were deeply anaesthetized with a mixture of
described by Destexhe et al. (1996). Here, ICAN is indirectly influenced
O2 and NO2 (2:1) and 5% halothane, a wide craniotomy was per-
by Ca2⫹ via a regulatory protein, P, which itself binds to the closed
formed, and the brain was removed. Coronal or sagittal slices of
form of the CAN channel, leading to channel opening (see Supple-
the thalamus containing the dorsal lateral geniculate nucleus (LGN)
were prepared and maintained as described previously (Williams et
DC1 for further details).
al., 1997), except for the presence of the cyclooxygenase inhibitor,
indomethacin (45 M) (Pakhotin et al., 1997), in the cutting solution.
Slices (400–500 m) were perfused with a warmed (35⬚C ⫾ 1⬚C),
continuously oxygenated (95% O2, 5% CO2) artificial cerebrospinal
This paper is dedicated to the late Dr. Rosario Samanin, Head of
fluid (ACSF) containing 134 mM NaCl, 2 mM KCl, 1.25 mM KH2PO4,
the Department of Neuroscience, Mario Negri Institute for Pharma-
1 mM MgSO4, 2 mM CaCl2, 16 mM NaHCO3, 10 mM glucose. For
cological Research, Milan, Italy. We wish to thank Dr. J.P. Turner
experiments involving NiCl2, CdCl2, or BaCl2, MgSO4 was replaced
for helpful advice on experiments, Drs. H.R. Parri and T.I. To´th for
with MgCl2 and KH2PO4 was omitted. Nifedipine was dissolved in
useful discussions on the manuscript, and Mr. T.M. Gould for techni-
100% ethanol and added to the final solution, such that the total
cal assistance. This work was supported by the Wellcome Trust
volume of ethanol did not exceed 0.1%. Experiments involving the
(grant 37089-98). Website: www.thalamus.org.uk.
addition of nifedipine were performed in semidarkness. All other
drugs were dissolved directly in ACSF. Drugs were obtained from the
Received: June 4, 2001
following sources: (RS)-1-aminoindan-1,5-dicarboxylic acid (AIDA),
Revised: January 14, 2002
valeric acid (APV), (⫹)-2-methyl-4-carboxyphenylglycine (LY367385),
P-(3-aminopropyl)-P-diethoxymetyl-phosphinic acid (CGP 56999A),
Achermann, P., and Borbe´ly, A. (1997). Low-frequency (⬍1 Hz) oscil-
lations in the human sleep EEG. Neuroscience 81, 213–222.
clopropa[b]chromen-1a-carboxylate ethyl ester (CPCCOEt),
Amzica, F., and Steriade, M. (1995). Disconnection of synaptic link-
ages disrupts synchronization of a slow oscillation. J. Neurosci. 15,
Amzica, F., and Steriade, M. (1997). The K-complex: its slow rhyth-
micity and relation to delta waves. Neurology 49, 952–959.
Bal, T., and McCormick, D.A. (1993). Mechanisms of oscillatory ac-
glutamic acid (EGLU), 2-methyl-6-(phenylethynyl)pyridine (MPEP),
tivity in guinea-pig nucleus reticularis thalami in vitro: a mammalian
pacemaker. J. Physiol. 468, 669–691.
midinium chloride (ZD 7288) were from Tocris-Cookson (UK);
4-aminopyridine (4-AP), bis-(o-aminophenoxy)-N,N,N⬘,N⬘,-tetraace-
Bal, T., and McCormick, D.A. (1996). What stops synchronized thala-
tic acid (BAPTA), bicuculline methiodide (BMI), ethylene glycol-bis
mocortical oscillations? Neuron 17, 297–308.
(b-aminoethyl ether)-N,N,N⬘,N⬘-tetraacetic acid (EGTA), and nifedi-
Chuang, S.C., Bianchi, R., and Wong, R.K. (2000). Group I mGluR
pine were from Sigma (UK).
activation turns on a voltage-gated inward current in hippocampal
pyramidal cells. J. Neurophysiol. 83, 2844–2853.
Contreras, D., and Steriade, M. (1995). Cellular basis of EEG slow
Extracellular recordings were performed using glass pipettes filled
rhythms: a study of dynamic corticothalamic relationships. J. Neu-
with 0.5 M NaCl (resistance: 1–5 M⍀) connected to a Neurolog
rosci. 15, 604–622.
104 differential amplifier (Digitimer, Welwyn Garden City, UK) and
Contreras, D., and Steriade, M. (1997a). Synchronization of low-
bandpass filtered at 0.1–20 kHz. Intracellular recordings, using the
frequency rhythms in corticothalamic networks. Neuroscience 76,
current clamp technique, were performed with standard wall glass
microelectrodes filled with 1 M potassium acetate (resistance: 80–
Contreras, D., and Steriade, M. (1997b). State-dependent fluctua-
120 M⍀), and in some cases 2% biocytin, and connected to an
tions of low-frequency rhythms in corticothalamic networks. Neuro-
Axoclamp-2A amplifier (Axon Instruments, Foster City, CA) op-
science 76, 25–38.
erating in bridge mode. Voltage clamp recordings were performed
using the single-electrode voltage clamp technique. During voltage
Contreras, D., Timofeev, I., and Steriade, M. (1996). Mechanisms of
clamp experiments, the headstage output was continuously moni-
long-lasting hyperpolarizations underlying slow sleep oscillations in
tored in order to ensure adequate settling time prior to current
cat corticothalamic networks. J. Physiol. 494, 251–264.
injection. Impaled cells were identified as TC neurons using estab-
Crunelli, V., Lightowler, S., and Pollard, C.E. (1989). A T-type Ca2⫹
lished electrophysiological and morphological criteria (Williams et
current underlies low-threshold Ca2⫹ potentials in cells of the cat
al., 1997). Voltage and current records were stored on a Biologic
and rat lateral geniculate nucleus. J. Physiol. 413, 543–561.
DAT recorder (IntraCel, Royston, UK) and later analyzed using
Destexhe, A., Bal, T., McCormick, D.A., and Sejnowski, T.J. (1996).
Clampfit (Axon Instruments). The apparent input resistance (RN) was
Ionic mechanisms underlying synchronized oscillations and propa-
calculated from the peak of voltage responses evoked at ⫺60 mV
gating waves in a model of ferret thalamic slices. J. Neurophysiol.
by small (20–50 pA) current steps. All quantitative results in the text
and figures are expressed as mean ⫾ SEM. Statistical significance
Godwin, D.W., Van Horn, S.C., Eriir, A., Sesma, M., Romano, C.,
was assessed using Student's t test. Electrical stimulation of corti-
and Sherman, S.M. (1996). Ultrastructural localization suggests that
cothalamic fibers was performed in saggital slices using a bipolar
retinal and cortical inputs access different metabotropic glutamate
tungsten electrode placed in the optic radiation (typically 25–100
receptors in the lateral geniculate nucleus. J. Neurosci. 16, 8181–
Hz for 100–500 ms, with each stimulus being a 0.2 ms pulse of
Gutierrez, C., Cox, C.L., Rinzel, J., and Sherman, S.M. (2001). Dy-
namics of low-threshold spike activation in relay neurons of the cat
Dynamic Clamp and Computational Modeling
lateral geniculate nucleus. J. Neurosci. 21, 1022–1032.
The dynamic clamp system was implemented as described pre-
Hughes, S.W., Cope, D.W., To´th, T.I., Williams, S.R., and Crunelli,
viously (Hughes et al., 1999). Computer simulations were performed
V. (1999). All thalamocortical neurones possess a T-type Ca2⫹ "win-
using a previously described biophysical model of a TC neuron that
dow" current that enables the expression of bistability-mediated
comprised the currents ILeak, IT, Ih, INa, and IKdr (i.e., a delayed rectifier)
activities. J. Physiol. 517, 805–815.
(Williams et al., 1997). A range of gLeak values (1.2–2.0 nS) was investi-
gated that reproduced a range of apparent input resistances that
Lee, K.H., and McCormick, D.A. (1997). Modulation of spindle oscil-
lations by acetylcholine, cholecystokinin and 1S,3R-ACPD in the
neurons of the rat dorsal lateral geniculate nucleus in vitro. Neurosci-
ferret lateral geniculate and perigeniculate nuclei in vitro. Neurosci-
ence 100, 493–505.
ence 77, 335–350.
von Krosigk, M., Monckton, J.E., Reiner, P.B., and McCormick, D.A.
Leresche, N., Lightowler, S., Soltesz, I., Jassik-Gerschenfeld, D.,
(1999). Dynamic properties of corticothalamic excitatory postsynap-
and Crunelli, V. (1991). Low-frequency oscillatory activities intrinsic
tic potentials and thalamic reticular inhibitory postsynaptic poten-
to rat and cat thalamocortical cells. J. Physiol. 315, 155–174.
tials in thalamocortical neurons of the guinea-pig dorsal lateral ge-
niculate nucleus. Neuroscience 91, 7–20.
Lu¨thi, A., and McCormick, D.A. (1998). Periodicity of thalamic syn-
Williams, S.R., Turner, J.P., To´th, T.I., Hughes, S.W., and Crunelli, V.
chronized oscillations: the role of Ca2⫹-mediated upregulation of Ih.
(1997). The "window" component of the low threshold Ca2⫹ current
Neuron 20, 553–563.
produces input signal amplification and bistability in cat and rat
McCormick, D.A., and Pape, H.C. (1990). Properties of a hyperpolar-
thalamocortical neurones. J. Physiol. 505, 689–705.
ization-activated cation current and its role in rhythmic oscillation
Zhan, X.J., Cox, C.L., and Sherman, S.M. (2000). Dendritic depolar-
in thalamic relay neurons. J. Physiol. 431, 291–318.
ization efficiently attenuates low-threshold calcium spikes in thala-
McCormick, D.A., and von Krosigk, M. (1992). Corticothalamic acti-
mic relay cells. J. Neurosci. 20, 3909–3914.
vation modulates thalamic firing through glutamate "metabotropic"
Zhu, J.J., Uhlrich, D., and Lytton, W.W. (1999). Burst firing in identi-
receptors. Proc. Natl. Acad. Sci. USA 89, 2774–2778.
fied rat geniculate interneurons. Neuroscience 91, 1445–1460.
Pakhotin, P.I., Pakhotina, I.D., and Andreev, A.A. (1997). Functional
stability of hippocampal slices after treatment with cyclooxygenase
inhibitors. Neuroreport 8, 1755–1759.
Partridge, L.D., and Swandulla, D. (1988). Calcium-activated non-
specific cation channels. Trends Neurosci. 11, 69–72.
Sanchez-Vives, M.V., and McCormick, D.A. (2000). Cellular and net-
work mechanisms of rhythmic recurrent activity in neocortex. Nat.
Neurosci. 3, 1027–1034.
Simon, N.R., Manshanden, I., and Lopes da Silva, F.H. (2000). A
MEG study of sleep. Brain Res. 860, 64–76.
Soltesz, I., Lightowler, S., Leresche, N., Jassik-Gerschenfeld, D.,
Pollard, C.E., and Crunelli, V. (1991). Two inward currents and the
transformation of low-frequency oscillations of rat and cat thalamo-
cortical cells. J. Physiol. 441, 175–197.
Steriade, M., Curro´ Dossi, R., and Nun˜ez, A. (1991). Network modula-
tion of a slow intrinsic oscillation of cat thalamocortical neurons
implicated in sleep delta-waves—cortically induced synchronization
and brainstem cholinergic suppression. J. Neurosci. 11, 3200–3217.
Steriade, M., Contreras, D., Curro´ Dossi, R., and Nun˜ez, A. (1993a).
The slow (⬍ 1 Hz) oscillation in reticular thalamic and thalamocortical
neurons: scenario of sleep rhythm generation in interacting thalamic
and neocortical networks. J. Neurosci. 13, 3284–3299.
Steriade, M., McCormick, D.A., and Sejnowski, T.J. (1993b). Thala-
mocortical oscillations in the sleeping and aroused brain. Science
262, 679–685.
Steriade, M., Nun˜ez, A., and Amzica, F. (1993c). A novel slow (⬍1 Hz)
oscillation of neocortical neurons in vivo. J. Neurosci. 13, 3253–3265.
Steriade, M., Nun˜ez, A., and Amzica, F. (1993d). Intracellular analysis
between slow (⬍1 Hz) neocortical oscillation and other sleep
rhythms of the electroencephalogram. J. Neurosci. 13, 3266–3283.
Steriade, M., Contreras, D., Amzica, F., and Timofeev, I. (1996).
Synchronization of fast (30–40 Hz) spontaneous oscillations in intra-
thalamic and thalamocortical networks. J. Neurosci. 16, 2788–808.
Steriade, M., Timofeev, I., and Grenier, F. (2001). Natural waking
and sleep states: a view from inside neocortical neurons. J. Neuro-
physiol. 85, 1969–85.
Tennigkeit, F., Schwarz, D.W., and Puil, E. (1999). Effects of metabo-
tropic glutamate receptor activation in auditory thalamus. J. Neuro-
physiol. 82, 718–729.
Timofeev, I., and Steriade, M. (1996). Low-frequency rhythms in the
thalamus of intact-cortex and decorticated cats. J. Neurophysiol.
76, 4152–4168.
Tokimasa, T. (1985). Intracellular Ca2⫹-ions inactivate K⫹-current in
bullfrog sympathetic neurons. Brain Res. 337, 386–391.
To´th, T.I., Hughes, S.W., and Crunelli, V. (1998). Analysis and bio-
physical interpretation of bistable behaviour in thalamocortical neu-
rons. Neuroscience 87, 519–523.
Turner, J.P., and Salt, T.E. (1998). Characterization of sensory and
corticothalamic excitatory inputs to rat thalamocortical neurones in
vitro. J. Physiol. 510, 829–843.
Turner, J.P., and Salt, T.E. (2000). Synaptic activation of the group
I metabotropic glutamate receptor mGlu1 on the thalamocortical
Source: ftp://coriandre.extra.cea.fr/pub/dsv/madic/concarneau03/intervenants/Lambert_et_Leresche/Hughes_et_al.,_2002.pdf
Neurofeedback – How Attention Takes Flight Pierre Walther and Stephan Ellinger Goethe University Frankfurt, Julius-Maximillians-University Würzburg (GERMANY) Attention Deficit Disorder (ADD) alone or in combination with Hyperactivity (ADHD) is one of the most common disorders in childhood and adolescence and even persists into adulthood. Children with ADHD show a higher amount of slow brain waves and a decreased amount of faster brain waves compared to children without ADHD (Barry et al., 2003). The basic idea of neurofeedback is to transfer the unconscious process of brain wave function into a conscious process by reporting it to the patient. The Brainfeeders project aims to evaluate the possibilities for integrating neurofeedback in a school setting. The primary goal of the study is to replicate results found in clinical trials without any additional human resources. We would like to evaluate how well a training programme like this fits in school settings and if results are comparable to clinical studies. We are interested in forming a transnational working group, integrating researchers who are working on similar projects or who are interested in working on Brainfeeders in their countries.
European Journal of Pain 10 (2006) 185–192 Guidelines for the use of antidepressants in painful rheumatic conditions Serge Perrot *, Emmanuel Maheu, Rose-Marie Javier, Alain Eschalier, Anne Coutaux, Manuela LeBars, Philippe Bertin, Bernard Bannwarth, Richard Tre ves Cercle dÕe´tude de la douleur en rhumatologie, CEDR, Limoges, France Received 31 August 2004; accepted 11 March 2005