For immunohistochemistry 4μm-thick wax section were deparaffinized through xylene
SAMP1 mice as a new animal model for photoaging
of the skin associated with spontaneous higher
oxidative stress status
Contents
General Introduction .….……………………………………………… 4
CHAPTER 1. Spontaneous occurrence of photoaging-like phenotypes in the
dorsal skin of old SAMP1 mice, an oxidative stress model
Materials and Methods .……………………………………………. 13
CHAPTER 2. Differences in the histopathology and cytokine expression
pattern between chronological aging and photoaging of hairless mice skin
Abstract ……………………………………………………. 40
Materials and Methods …………………………………………. 44
ANOVA, analysis of variance
DEJ, dermal-epidermal junction
GAG, glycosaminoglycan
H&E, hematoxylin-eosin
IFN-γ, interferon-gamma
IL-1β, interleukin-1 beta
IL-6, interleukin-6
iNOS, inducible nitric oxide synthase
MMP, matrix metalloproteinase
PBS, phosphate-buffered saline
ROS, reactive oxygen species
SAMP, senescence-accelerated mouse-prone
SAMR, senescence-accelerated mouse-resistant
TBARS, thiobarbituric acid reactive substances
TGF-β1, transforming growth factor-beta 1
TNF-α, tumor necrosis factor-alpha
General Introduction
A major function of skin is to protect organisms from physical and
environmental assaults such as solar radiation, infection, or dehydration. These
protective functions can decline with age [1]. Aging of the skin is a process in
which both intrinsic and extrinsic determinants lead to a progressive loss of
structural integrity and physiological function [2]. The intrinsic aging process is
characterized by slow and irreversible tissue degeneration, and affects the skin as
well as the whole body. The extrinsic aging process, i.e. "photoaging", is
provoked by chronic exposure to sunlight, and especially ultraviolet (UV) light
[1,3]. In short, photoaging refers to the effects of long-term UV exposure [1].
Since people are living longer, and spend more spare time outdoors, this leads to
excessive exposure to UV radiation from natural sunlight, resulting in an ever
increasing demand to protect human skin against the detrimental effects of UV
exposure [4]. Photoaged skin is clinically important not only because it causes
considerable cosmetic and psychosocial distress in older people, but also because
benign, premalignant, and malignant neoplastic growths flourish in photoaged
UV radiation consists of UVA (320 to 400 nm), UVB (280 to 320 nm) and
UVC (200 to 280 nm). More than 90% of UV radiation that reaches the earth is
UVA which penetrates deep into the epidermis and dermis of the skin. Compared
to UVB, UVA is more effective in the production of an immediate tanning effect
[7]. Although UVB is a minor component, it is the most active constituent of
solar light. UVB is 1000 times more capable of causing sunburns and more
genotoxic than UVA [7]. The third type of UV radiation, UVC, also called
shortwave or ionizing radiation, is absorbed by gas in the atmosphere, and thus
does not reach the earth's surface and therefore does not normally contribute to
photodamage [8]. As for the pathogenesis of photoaging, reactive oxygen species
(ROS) generated by UV radiation are thought to play an integral role.
UV-induced ROS can exert a multitude of effects such as lipid peroxidation, the
activation of transcription factors and the generation of DNA strand breaks [1].
Moreover, UV radiation stimulates and activates various cells to produce and
release pro-inflammatory cytokines and matrix metalloproteinases (MMPs) that
may play important roles in the photoaging process [9].
The most conspicuous stigmata of skin aging appear on sun-exposed areas
in the elderly, especially on the face, and results in photoaging. Photoaged skin is
histologically characterized by elastosis [10], which generally begins at the
junction of the papillary and reticular dermis. Other histological changes
characterizing photoaged skin include a large increase in the deposition of
glycosaminoglycan (GAG) [11,12], fragmented elastic fibers [13], and the
formation of the Grenz zone [3], which is a narrow band in the uppermost portion
of the dermis composed primarily of GAGs and newly formed collagen [14].
The long latency period and slow evolution of photoaging make human
studies difficult [3]. Therefore, the development of a reliable animal model is
necessary to systematically study the pathogenesis of photoaged skin. At present,
UV-irradiated skh-hairless mice are widely used as an animal model for skin
photoaging [15]. In this model, UV irradiation generally starts at 6 to 8 weeks of
age, and is continued for 10 to 22 weeks to produce photoaging-like skin lesions
[16,17]. This model mimics the extrinsic aspects of the pathogenesis of
photoaging, and recapitulates many features of human photoaging. However,
human photoaging is considered to be the superposition of solar damage on the
normal aging process [2], and it is difficult to study the contribution of the
intrinsic aging process in the pathogenesis of photoaging using this model. It is
important to elucidate how the intrinsic aging process contributes to the
pathogenesis of photoaging
in vivo, since intrinsic factor(s) seems to be essential
for the manifestation of skin photoaging phenotypes in humans.
In the present study, I describe senescence-accelerated mouse-prone 1
(SAMP1) strain of mice with higher oxidative status, as a new animal model for
human skin photoaging due to exaggerated intrinsic factors. This mouse may be a
useful model to study the contribution of intrinsic aging processes in the
pathogenesis of photoaging. Furthermore, I compared the histological changes
and cytokine expression patterns between UV-irradiated hairless mice, a standard
photoaging model, and non-irradiated, chronologically aged hairless mice to
clarify factor(s) that differentiate photoaging from chronological aging
phenotypes in the skin.
Finally, an imbalance between pro-inflammatory and anti-inflammatory
conditions caused by UV and/or ROS is proposed as a possible pathogenetic
factor of the skin photoaging.
CHAPTER 1
Spontaneous occurrence of photoaging-like phenotypes in the
dorsal skin of old SAMP1 mice, an oxidative stress model
Abstract
I showed that skin from old senescence-accelerated mouse-prone 1
(SAMP1) mice, a model for accelerated senescence and higher oxidative status,
exhibited histological and gene expression changes similar to those in human
photoaged skin without UV irradiation. Histopathological analysis revealed an
age-associated increase in the elastic fiber and glycosaminoglycan content of the
dermis of 48- to 70-week-old SAMP1 mice. I observed an upregulation of
several pro-inflammatory cytokines and matrix metalloproteinases-7 and -12
with advancing age in SAMP1 skin. These changes occurred concomitantly with
an increase in lipid peroxide levels in the skin. These age-associated changes
were not observed in skin from control (long-lived) senescence-accelerated
mouse-resistant 1 (SAMR1) mice. I propose that SAMP1 mice are a
spontaneous animal model for human photoaging caused by an exaggerated
intrinsic mechanism, which is useful to explore the link between oxidative stress
and photoaging, and to evaluate the efficacy of antioxidants.
As mentioned in the General Introduction, chronic exposure to sunlight is
required to develop characteristic stigmata observed in aging skin. Human skin
photoaging can be considered to be a superposition of solar damage on the
normal aging process [2]. The long latency period and slow evolution of
photoaging makes human studies difficult [3]. Therefore, the development of a
reliable animal model is necessary to systematically study the pathogenesis of
photoaged skin. UV-irradiated skh-hairless mice are widely used as a photoaging
model [15]. This model recapitulates many features of human photoaging.
However, the aforementioned animal model only mimics the extrinsic aspects of
the pathogenesis of photoaging, and the contribution of the intrinsic aging
process is difficult to study using this model. As described in the General
Introduction, reactive oxygen species (ROS) generated by UV are suspected to be
important factors for the pathogenesis of photoaging. ROS are also important
factors for senescence and/or age-associated degenerative changes in various
tissues [18,19].
The senescence-accelerated mouse (SAM), a group of related inbred strains,
was developed as a model of senescence acceleration and geriatric disorders
observed in humans [20-23]. These mice consist of a series of SAMP
(accelerated senescence-prone, short-lived) and SAMR (accelerated
senescence-resistant, long-lived) strains. SAMP mice show an accelerated
senescence process, shorter lifespan and an earlier onset and more rapid
progression of age-associated pathological phenotypes when compared to SAMR
mice [23,24]. Spontaneous higher oxidative stress status due to mitochondrial
dysfunction is thought to be a contributing factor to senescence acceleration and
age-associated pathologies in SAMP mice (reviewed in 18,19). Hosokawa
et al.,
revealed that murine dermal fibroblast-like (MDF) cells from dorsal dermis of
neonatal SAMP11 mice showed earlier occurrence of senescence/crisis than those
from SAMR1 mice [25]. MDF cells from SAMP11 mice showed higher lipid
peroxide (LPO) contents than those from SAMR1 mice [26]. Furthermore,
supplementation of media with aminoguanidine, an inhibitor of diamine oxidase
and nitric oxide synthase, delayed the senescence/crisis and decreased the LPO
content in MDF cells from SAMP11 mice to levels found in those from SAMR1
mice [26]. These data suggest that higher oxidative stress in SAMP11 cells may
contribute to accelerated senescence
in vitro. An increase in the production of
ROS from MDF cells from SAMP11 mice was also confirmed by live cell
imaging, and the main source of ROS production was mitochondria [23].
Mitochondrial function and ultrastructures were also impaired in MDF cells from
SAMP11 mice when compared with those from SAMR1 mice [23].
Several studies have addressed aging changes in the skin of SAMP mice.
Changes in the levels of thiobarbituric acid reactive substances (TBARS) occur at
middle age, and histological changes at a later age in SAMP1 skin [27]. Okada
et
al., reported that the deposition of elastic fibers was observed in 12- to
14-month-old SAMP1 skin [28]. After these reports, however, there has been no
systematic study to investigate aging changes of SAMP1 skin.
On the basis of these previous studies, I hypothesized that the age-associated
skin changes of SAMP1 mice may be similar to photoaged skin. The present
study was undertaken to characterize the morphological and biochemical aspects
of age-associated changes in the skin from SAMP1 mice, and to test the validity
of SAMP1 mice as an animal model for human skin photoaging.
Materials and Methods
SAMP1 and SAMR1 strains of mice were bred under conventional
conditions, housed at 23 ± 2ºC, and allowed free access to food (CE2, Nihon
CLEA, Tokyo, Japan) and tap water. The light-dark cycle was set at 12 hours
(lights were on at 07:00). In SAMP1 mice, the mean life span for the last one
tenth of a group of survivors for males was 535 days, and the median survival
time for males was 361 days. In SAMR1 mice, which have a normal life span,
the mean life span for the last one tenth of a group of survivors for males was
783 days, and the median survival time for males was 634 days [29]. Male
SAMP1 mice at 12, 24, 36, 48, 56 and 70 weeks of age and SAMR1 mice at 12
and 70 weeks of age were used. I checked all mice pathologically after sampling
the skin specimens, and excluded those samples from mice with
inflammation-associated phenotypes (pneumonia and colitis) and/or tumors
from the subsequent analyses.
I took special care to minimize the number of animals used and their
suffering. All animals were handled in accordance with the Guide for the Care
and Use of Laboratory Animal of the Institute for Developmental Research,
Aichi Human Service Center.
Histology
Each mouse was sacrificed by cervical dislocation, and the dorsal skin was
rapidly removed, immersed in 10% neutral buffered formalin (pH 7.4) for 7 days,
and embedded in paraffin. Twenty-micron-thick sections were cut with a sliding
microtome, and hematoxylin and eosin (H&E), toluidine blue, resorcin-fuchsin
and alcian blue staining procedures were performed according to standard
The density of mast cells was evaluated using sections stained with
toluidine blue. Photomicrographs of continuous, non-overlapping visual fields
(585 × 424 μm) were obtained to cover the entire dermis of the specimen using a
digital microscope (VHX-200, Keyence Corporation, Osaka, Japan). The mast
cells were counted, and the density was calculated by dividing the mast cell
number by the evaluated area.
RNA extraction and real-time quantitative PCR
The dorsal skin was dissected from each mouse and washed in ice-cold
phosphate-buffered saline (PBS). The total RNA was isolated using an ISOGEN
kit (NIPPON GENE CO., LTD., Tokyo, Japan) according to the manufacturer's
instructions. The RNA yields and purities were determined by
spectrophotometric absorption analyses at 260/280 nm. cDNA was synthesized
from the total RNA using a SuperScriptTM III First-Strand Synthesis System for
RT-PCR (Invitrogen, Carlsbad, CA, USA) according to the manufacturer's
Gene expression was analyzed by a real time RT-PCR system (ABI
Stepone-Plus, Applied Biosystems, Foster City, CA, USA) with each cDNA
sample, specific Taqman primers/probes and a Taqman Universal PCR Master
Mix (Applied Biosystems). The following probes were used (identified by
Applied Biosystems assay identification number): IL-1 beta (IL-1β),
Mm00434228_m1; TNF-alpha (TNF-α), Mm00443258_m1; IL-6,
Mm00446190_m1; IFN-gamma (IFN- γ), Mm00801778_m1; TGF-beta1
(TGF-β1), Mm00441724_m1; iNOS, Mm00440485_m1; IL-4,
Mm00445260_m1; MMP7, Mm001168420_m1; MMP-12, Mm00500554_m1
and 18S rRNA, Hs99999901_s1. 18S rRNA was used as an internal control. The
expression of these mRNA species was analyzed using the same sets of total
RNA samples. The reaction mixtures were subjected to the following
amplification scheme: 1 cycle at 50ºC for 2 min (AmpErase
uracil-N-glycosylase deactivation) and 1 cycle at 95ºC for 10 min (AmpliTaq
Gold activation), followed by 40 cycles at 95ºC for 15 s (denaturation) and 60ºC
for 1 min (annealing and extension).
Measurement of skin thiobarbituric acid reactive substances (TBARS)
The TBARS levels in the skin were measured as a marker of lipid
peroxidation [30] using the NWLSSTM Malondialdehyde Assay (Northwest Life
Science Specialties, Vancouver, WA, USA). The dorsal skin was removed,
frozen in liquid nitrogen and then homogenized with a mortar. The homogenate
was mixed with the assay buffer from the kit, mixed vigorously and centrifuged
at 10,000 × g for 5 min. The supernatant or standards (250 μl) were added to test
tubes containing 10 μl of butylated hydroxytoluene. After the addition of 250 μl
of 1 M phosphoric acid and 250 μl of thiobarbituric acid solution, the tubes were
mixed vigorously and incubated for 60 min at 60ºC. The mixture was then
centrifuged at 10,000 × g for 3 min, and the absorbance spectra were recorded
between 400 nm and 700 nm using a spectrophotometer (Ultrospec 2000,
Pharmacia Biotech, Cambridge, UK), and the TBARS levels were determined
according to the manufacturer's recommendations. The protein concentration in
the sample was determined by the Bradford assay using bovine serum albumin
as the standard.
Statistical Analyses
The data were expressed as means ± S.D. A one-way analysis of variance
(ANOVA) followed by Tukey's procedure were performed to determine the
differences in the expression of mRNA and skin TBARS levels among different
age groups of SAMP1 mice. A two-way ANOVA, followed by a simple main
effects analysis was performed to examine the difference in the expression of
mRNA and the skin TBARS levels among 12- and 70-week-old SAMP1 and
SAMR1 mice. The number of mast cells between 12- and 70-week-old SAMP1
mice was compared using Student's t-test. Differences were considered to be
statistically significant when p < 0.05.
Histological analyses of age-associated changes in SAMP1 versus SAMR1
H&E staining revealed an increase in the area of flattened
dermal-epidermal junctions (DEJ), in the number of immature fibroblasts
beneath the epidermis, and in the epidermal thickness at 56 and 70 weeks of age
in SAMP1 mice (Fig. 1e, f). Irregularity in the direction of hair shaft growth
(Fig. 1e, f) and the formation of Grenz zones (Fig. 1e, f, asterisks) were
observed in 56- and 70-week-old SAMP1 mice. In contrast, 70-week-old
SAMR1 mice did not exhibit any significant age-related changes in the skin
when compared to 12-week-old SAMR1 mice (Fig. 1g, h).
Toluidine blue staining revealed that the number of infiltrating mast cells
was increased in the dermis of SAMP1 mice at 70 weeks of age. The number of
mast cells at 70 weeks of age was 3.1-fold greater than that at 12 weeks of age
(267.3 ± 172.4 vs. 86.0 ± 7.84/mm2, p = 0.047). In contrast, 70-week-old
SAMR1 mice did not show this increase in dermal mast cells (data not shown).
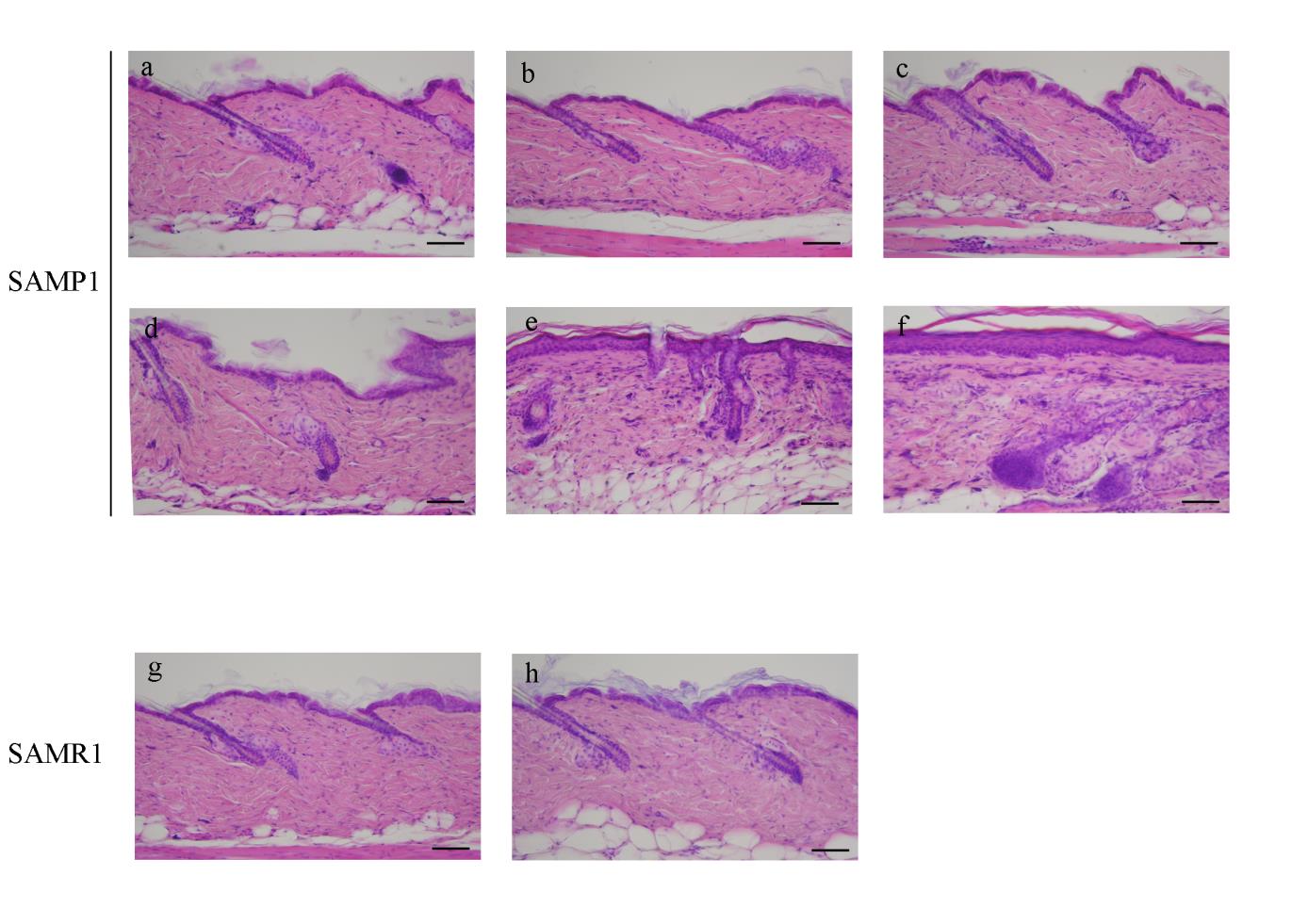
Fig. 1. Histological changes in SAMP1 and SAMR1 mouse dorsal skin revealed by
hematoxylin and eosin (H&E) staining.
Representative photomicrographs of the dorsal skin from 12- (a), 24- (b), 36- (c), 48- (d), 56-
(e) and 70-week-old (f) SAMP1 mice and 12- (g) and 70-week-old (h) SAMR1 mice.
Formalin-fixed skin sections were stained with H&E. Asterisk in e and f indicates Grenz zone.
See the text for the detailed description. Scale bars: 100 μm.
Using resorcin-fuchsin staining, numerous fine and highly branched elastic
fibers were deposited in the dermis of SAMP1 mice, which first appeared at 48
weeks of age (Fig. 2d), and increased until 70 weeks of age (Fig. 2e, f) to
develop overt elastosis (Fig. 2f, open arrowhead). In SAMR1 mice,
resorcin-fuchsin staining did not show any age-related changes in the dermal
elastic fibers (Fig. 2g, h).
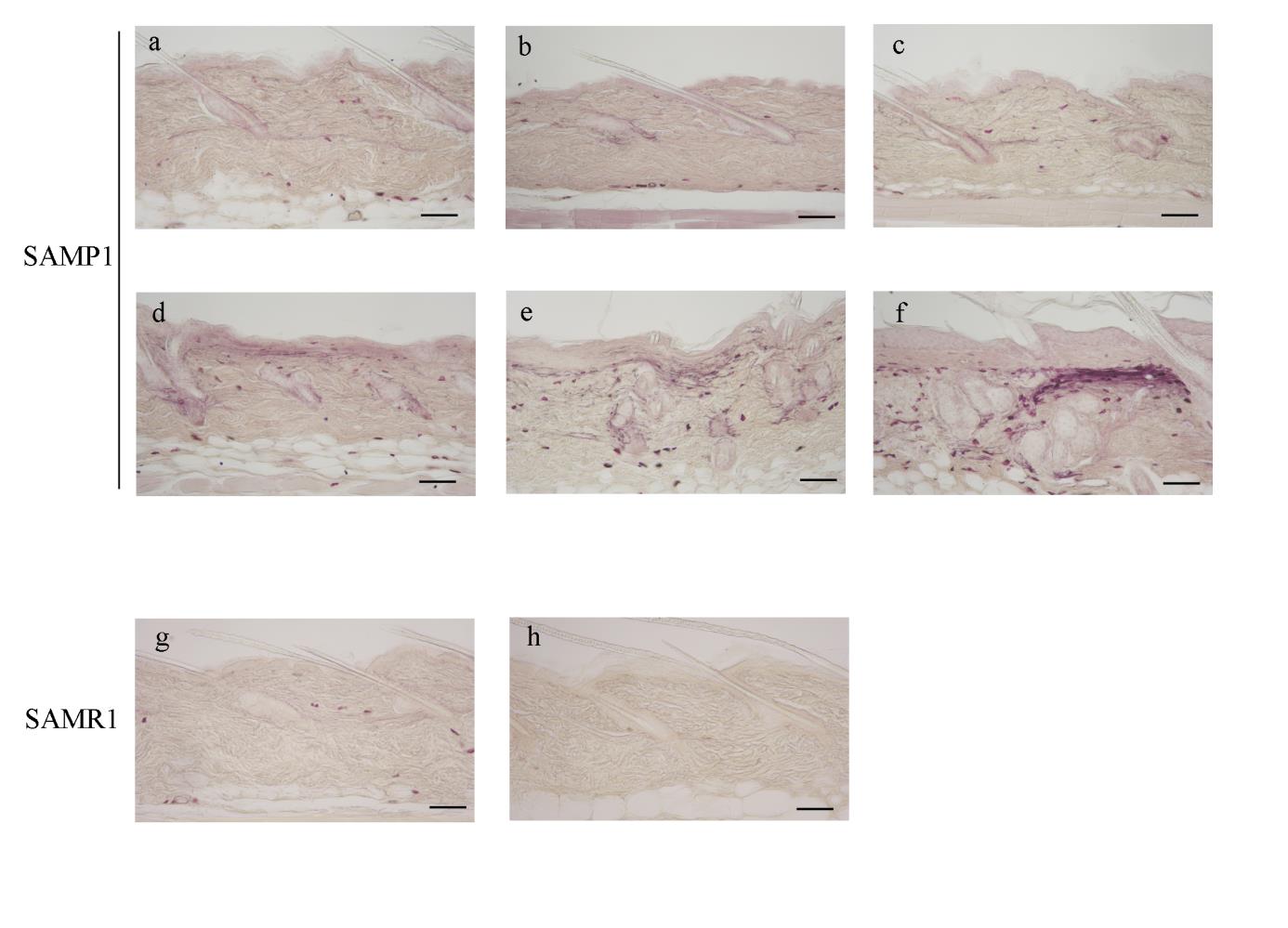
Fig. 2. Histological changes in SAMP1 and SAMR1 mouse dorsal skin revealed by
resorcin-fuchsin staining.
Representative photomicrographs of the dorsal skin from 12- (a), 24- (b), 36- (c), 48- (d), 56-
(e) and 70-week-old (f) SAMP1 mice and 12- (g) and 70-week-old (h) SAMR1 mice.
Formalin-fixed skin sections were stained with resorcin-fuchsin The open arrowhead in f
indicates overt elastosis in the dermis. See the text for the detailed description. Scale bars: 100 μm.
In alcian blue-stained sections, a prominent accumulation of alcian
blue-positive GAGs was observed just beneath the epidermis at 48 weeks of age
in SAMP1 mice (Fig. 3d, closed arrowheads), and the GAG-positive area spread
toward the deep layer of the dermis with advancing age (Fig. 3e, f). Again,
70-week-old SAMR1 mice did not show any changes in the GAG volume in
their dermis when compared to 12-week-old SAMR1 mice (Fig. 3g, h).
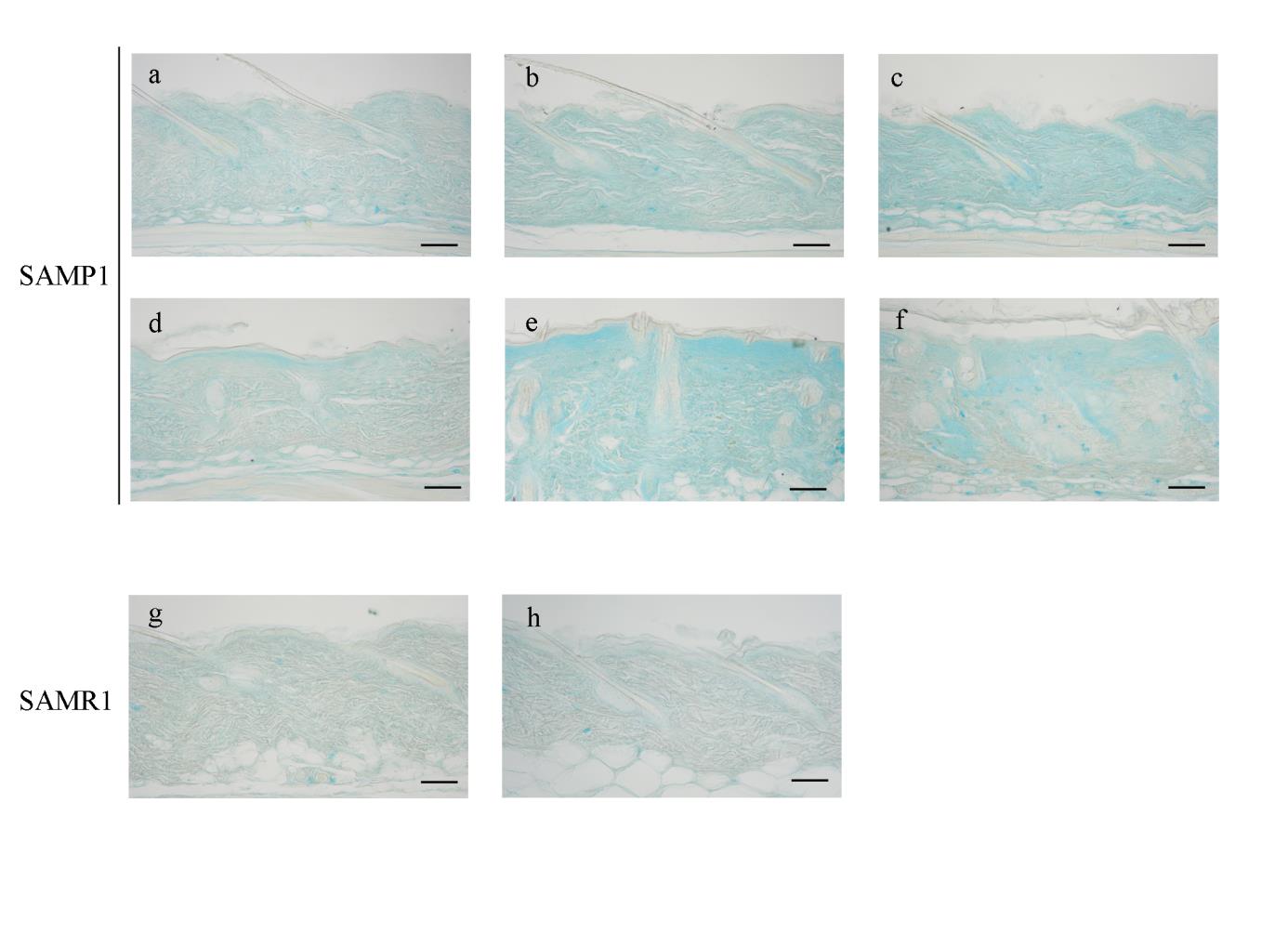
Fig. 3. Histological changes in SAMP1 and SAMR1 mouse dorsal skin revealed by alcian
Representative photomicrographs of the dorsal skin from 12- (a), 24- (b), 36- (c), 48- (d), 56-
(e) and 70-week-old (f) SAMP1 mice and 12- (g) and 70-week-old (h) SAMR1 mice.
Formalin-fixed skin sections were stained with alcian blue. The closed arrowheads in d
indicate increased glycosaminoglycans. See the text for the detailed description. Scale bars:
Gene expression analyses of SAMP1 and SAMR1 skin by real-time PCR
The expression of IL-1β mRNA tended to change with age in SAMP1 mice
(F(5,28) = 2.482, p = 0.0556). The expression of IL-1β mRNA peaked at 56
weeks of age, but then decreased at 70 weeks of age. A two-way ANOVA
showed a significant interaction between the effects of strain and age on the
expression of IL-1β (F(1,14) = 14.475, p = 0.0019). Simple main effects analysis
revealed that 70-week-old SAMP1 mice showed greater expression than that in
SAMR1 mice at the same age, whereas there were no differences between
12-week-old SAMP1 and SAMR1 mice (Fig. 4a).
In the expression of TNF-α mRNA, a one-way ANOVA showed a
significant effect for age in SAMP1 mice (F(5,32) = 8.703, p < 0.0001). The
TNF-α mRNA levels of 70-week-old SAMP1 mice were 12.9-fold greater than
that of 12-week-old SAMP1 mice. A two-way ANOVA showed a significant
interaction between the effects of strain and age on the expression of TNF-α
(F(1,17) = 4.701, p = 0.0446). Simple main effects analysis revealed that
70-week-old SAMP1 mice showed greater expression than that in SAMR1 mice
at the same age, whereas there were no differences between 12-week-old
SAMP1 and SAMR1 mice (Fig. 4b).
Fig. 4. Expression changes in IL-1β and TNF-α mRNA in the skin from SAMP1 and SAMR1
The expression of IL-1β (a) and TNF-α (b) mRNAs in the skin from SAMP1 and SAMR1
mice were analyzed by real-time PCR. The relative levels of mRNA were obtained by
dividing the quantity of each mRNA by that of 18S rRNA as the internal standard. The closed
bars represent SAMP1 mice, and the open bars represent SAMR1 mice. All data are presented
as means ± S.D. The number of mice used in this experiment ranged from 4 to 11 for each
experimental group. (*) p < 0.05, (**) p < 0.01.
In the expression of IL-6 mRNA, a one-way ANOVA showed a significant
effect for age in SAMP1 mice (F(5,31) = 3.317, p = 0.0163). The expression of
IL-6 mRNA increased dramatically at 56 weeks of age, and then decreased at 70
weeks of age. The expression level of IL-6 mRNA at 56 weeks of age was
6.4-fold higher than that at 12 weeks of age. In a two-way ANOVA, there were
no significant interaction between strain and age on the expression of IL-6
(F(1,15) = 2.776, p = 0.116) (Fig. 5a).
In the expression of IFN-γ mRNA, a one-way ANOVA showed a
significant effect for age in SAMP1 mice (F(5,30) = 5.605, p = 0.0009). The
expression of IFN-γ mRNA increased dramatically at 48 weeks of age, and
remained high until 70 weeks of age. The mRNA level at 70 weeks of age was
60.7-fold higher than that at 12 weeks of age. A two-way ANOVA showed a
significant interaction between the effects of strain and age on the expression of
IFN-γ (F(1,15) = 4.680, p = 0.0471). Simple main effects analysis revealed that
70-week-old SAMP1 mice showed greater expression than that in SAMR1 mice
at the same age, whereas there were no differences between 12-week-old
SAMP1 and SAMR1 mice (Fig. 5b).
Fig. 5. Expression changes in IL-6 and IFN-γ mRNA in the skin from SAMP1 and SAMR1
The expression of IL-6 (a) and IFN-γ (b) mRNAs in the skin from SAMP1 and SAMR1 mice
were analyzed by real-time PCR. The relative levels of mRNA were obtained by dividing the
quantity of each mRNA by that of 18S rRNA as the internal standard. The closed bars
represent SAMP1 mice, and the open bars represent SAMR1 mice. All data are presented as
means ± S.D. The number of mice used in this experiment ranged from 4 to 11 for each
experimental group. (*) p < 0.05, (**) p < 0.01.
In the expression of TGF-β1, a one-way ANOVA showed a significant
effect for age in SAMP1 mice (F(5,36) = 2.737, p = 0.0339). The expression
level of TGF-β1 mRNA at 70 weeks of age was 2.8-fold higher than that at 12
weeks of age. In a two-way ANOVA, there were no significant interaction
between strain and age on the expression of TGF-β1 (F(1,19) = 0.478, p =
0.498) (Fig. 6a).
In the expression of iNOS mRNA, a one-way ANOVA showed a
significant effect for age in SAMP1 mice (F(5,37) = 5.931, p = 0.0004). The
expression of iNOS mRNA increased gradually with age, and the mRNA level
at 70 weeks of age was 4.1-fold higher than that at 12 weeks of age. A two-way
ANOVA showed a trend for positive interaction on the expression of iNOS
(F(1,23) = 3.750, p = 0.0652) mRNA. The iNOS expression of 70-week-old
SAMP1 mice showed a greater expression of these mRNA than SAMR1 mice at
the same age (Fig. 6b).
Fig. 6. Expression changes in TGF-β and iNOS mRNA in the skin from SAMP1 and SAMR1
The expression of TGF-β1 (a) and iNOS (b) mRNAs in the skin from SAMP1 and SAMR1
mice were analyzed by real-time PCR. The relative levels of mRNA were obtained by
dividing the quantity of each mRNA by that of 18S rRNA as the internal standard. The closed
bars represent SAMP1 mice, and the open bars represent SAMR1 mice. All data are presented
as means ± S.D. The number of mice used in this experiment ranged from 4 to 11 for each
experimental group. (*) p < 0.05, (**) p < 0.01.
In the expression of MMP-7 mRNA, a one-way ANOVA showed a
significant effect for age in SAMP1 mice (F(5,47) = 5.285, p = 0.0007). The
expression of MMP-7 mRNA was very low until 48 weeks of age, and then
increased at older ages. The mRNA level at 70 weeks of age was 16.2-fold
higher than that at 12 weeks of age. A two-way ANOVA showed a significant
interaction between the effects of strain and age on the expression of MMP-7
(F(1,22) = 4.963, p = 0.0364) mRNA. Simple main effects analysis revealed that
70-week-old SAMP1 mice showed greater expression of these mRNA than
SAMR1 mice at the same age, whereas there were no differences between
12-week-old SAMP1 and SAMR1 mice (Fig. 7a).
In the expression of MMP-12 mRNA, a one-way ANOVA showed a
significant effect for age in SAMP1 mice (F(5,27) = 8.584, p < 0.0001). The
expression of MMP-12 mRNA increased dramatically at 48 weeks of age, and
peaked at 70 weeks of age. The expression level at 70 weeks of age was 4.7-fold
higher than that at 12 weeks of age. In a two-way ANOVA, there were no
significant interaction between strain and age on the expression of MMP-12
(F(1,17) = 0.036, p = 0.852) mRNA (Fig. 7b).
Fig. 7. Expression changes in MMP-7 and MMP-12 mRNA in the skin from SAMP1 and
The expression of MMP-7 (a) and MMP-12 (b) mRNAs in the skin from SAMP1 and
SAMR1 mice were analyzed by real-time PCR. The relative levels of mRNA were obtained
by dividing the quantity of each mRNA by that of 18S rRNA as the internal standard. The
closed bars represent SAMP1 mice, and the open bars represent SAMR1 mice. All data are
presented as means ± S.D. The number of mice used in this experiment ranged from 4 to 11
for each experimental group. (*) p < 0.05, (**) p < 0.01. N.D.: not detected.
Age-associated changes in the TBARS content in SAMP1 and SAMR1 skin
The extent of lipid peroxidation in the skin was assessed by measuring the
TBARS level. A one-way ANOVA showed a significant effect for age in
SAMP1 mice (F(5,27) = 5.890, p = 0.0008). The TBARS content peaked at 70
weeks of age. The TBARS level at 70 weeks of age was 2.6-fold greater than
that at 12 weeks of age. A two-way ANOVA showed a significant interaction
between the effects of strain and age on the TBARS content (F(1,15) = 11.044, p
= 0.0046). Simple main effects analysis showed that 70-week-old SAMP1 mice
showed greater TBARS content than SAMR1 mice at the same age, whereas
there were no differences between 12-week-old SAMP1 and SAMR1 mice (Fig.
Fig. 8. Age-associated changes in the TBARS levels in the skin from SAMP1 and SAMR1
The TBARS levels in the skin from SAMP1 and SAMR1 mice were measured and
normalized against the protein content of the homogenate. The closed bars represent SAMP1
mice, and the open bars represent SAMR1 mice. All data are presented as means ± S.D. The
number of mice used in this experiment ranged from 4 to 11 for each experimental group. (*)
p < 0.05, (**) p < 0.01
Discussion
In the present study, I demonstrated that the age-associated histological and
molecular expression changes in SAMP1 skin resembled those observed in
human photoaged skin. Table 1 summarizes the histological changes in old
SAMP1 skin, with reference to the histological characteristics of human
photoaged and intrinsically aged skin. The histological changes in old SAMP1
mice share many features with human photoaging, rather than with intrinsic
aging. In addition, skin from UV-irradiated hairless mice also exhibited similar
histological changes [3].
Recent studies have revealed that UV irradiation to human skin induces
transcriptional factor activator protein 1 (AP-1) and nuclear factor-kappa B
(NF-κB) upregulation [9]. AP-1 and NF-κB stimulate the transcription of genes
for matrix-degrading enzymes such as metalloproteinase and pro-inflammatory
cytokine genes, respectively [9]. The expression changes of these genes are
considered to be directly related to the pathogenesis of photoaged skin [31]. As
presented in Fig. 4 to 7 and Table 2, the age-associated changes in the
expression of these photoaging-associated molecules in SAMP1 skin were
similar to those observed in human photoaged skin. The upregulation of
pro-inflammatory cytokines, such as IL-1β [32,33], TNF-α [34], IL-6 [35,36]
and IFN-γ [37], has been described in human photoaged skin. The
age-associated upregulation of these cytokines has also been reported in the
brain of old SAMP8 [38] and SAMP10 [29] mice. Thus, a shift to a
pro-inflammatory status with advancing age may be a common phenomenon in
the SAMP strains of mice. The enhanced expression of TGF-β1 has been
reported in UVB- and solar-simulated UV-exposed skin [39,40]. iNOS mRNA
has been reported to be induced by UV exposure in human skin organ culture
[41]. Nitric oxide, produced by iNOS, reacts with superoxide to form
peroxinitrite, which then causes nitrosative damage to macromolecules. In fact,
3-nitrotyrosine is elevated in human photoaged skin [42]. MMPs are the main
contributor to the alterations in the composition of the dermal matrix, which are
the most conspicuous histological features of photoaged skin [1]. In the present
study, I focused on MMP-7 and -12, since I found a prominent increase in
elastic fibers in old SAMP1 skin. These MMPs have the capability to degrade
elastin, and are considered to contribute to the remodeling of the elastotic areas
in sun-damaged skin [43]. I found that both MMPs showed an age-associated
upregulation in SAMP1 skin (Fig. 7), which is a common feature with human
photoaged skin [43,44].
UV-induced ROS have been suggested to be a promoting factor for
photoaging [9], and chronic UV exposure leads to increased levels of
mitochondrial DNA deletions in human skin [45]. The results in this study
suggest that the increase in ROS production may be sufficient to induce
photoaging-like phenotypes. SAMP1 mice strains have been used in numerous
studies as a model for human geriatric disorders, and Hosokawa et al. previously
proposed that they are a model of a spontaneously higher oxidative status, partly
caused by mitochondrial dysfunction [19,24]. Increased TBARS levels in old
SAMP1 skin (Fig. 8), and increased ROS production in dermal fibroblast-like
cells from SAMP11 mice [23] indicate that the skin of old SAMP mice is under
a higher oxidative environment. UV-induced ROS contribute to the pathogenesis
of photoaging in humans, whereas ROS from defective mitochondria may be the
culprit responsible for the aging phenotypes of old SAMP1 mice. Considering
that ROS are at least a promoting factor for intrinsic aging, "extrinsic"
photoaging might be explained as an exaggerated form of intrinsic aging.
In summary, the skin from old SAMP1 mice exhibited phenotypes closely
resembling human photoaged skin, in terms of histological and molecular
expression changes and increased oxidative stress. SAMP1 mice may be a useful
spontaneous animal model for investigating the link among the intrinsic aging
process, oxidative stress, and photoaging phenotypes, and in evaluating the
efficacy of anti-photoaging therapy by topical and oral antioxidants [46].
Anatomic comparison between the dorsal skin from old SAMP1 mice, human photoaged skin
and intrinsically aged skin.
Dorsal skin from
photoaged skin a
Glycosaminoglycan
Markedly increased
Markedly increased
Slightly increased
Markedly increased;
Markedly increased;
degenerates into
degenerates into
Dermal-epidermal junction
Epidermal thickness
aModified from Refs. [2] and [3].
Changes in mRNA expression in the dorsal skin from old SAMP1 mice and human photoaged
Dorsal skin from
CHAPTER 2
Differences in the histopathology and cytokine expression pattern
between chronological aging and photoaging of hairless mice skin
Abstract
Skin photoaging is a complex, multifactorial process resulting in functional
and structural changes of the skin, and different phenotypes from chronological
skin aging are well-recognized. Ultraviolet (UV)-irradiated hairless mice have
been used as a skin photoaging animal model. However, differences in
morphology and gene expression patterns between UV-induced and
chronological skin changes in this mouse model have not been fully elucidated.
Here I investigated differences in histopathology and cytokine expression
between UV-irradiated and non-irradiated aged hairless mice to clarify factor(s)
that differentiate photoaging from chronological skin aging phenotypes.
Eight-week-old HR-1 hairless mice were divided into UV-irradiated
(UV-irradiated mice) and non-irradiated (control mice) groups. Irradiation was
performed three times per week for 10 weeks. In addition, 30-week-old HR-1
hairless mice were reared until 70 weeks of age without UV irradiation (aged
mice). Histopathologies revealed that the flattening of dermal-epidermal
junctions and epidermal thickening were observed only in UV-irradiated mice.
Decreases in fine elastic fibers just beneath the epidermis, the thickening of
elastic fibers in the reticular dermis, and the accumulation of
glycosaminoglycans were more prominent in UV-irradiated mice as compared
to non-irradiated aged mice. Quantitative PCR analyses revealed that
UV-irradiated mice showed an increase in the expression of IFN-γ. In contrast,
aged mice exhibited proportional upregulation of both pro-inflammatory and
anti-inflammatory cytokines. The IFN-γ/IL-4 ratio, an indicator for the balance
of pro-inflammatory and anti-inflammatory cytokines, was significantly higher
in UV-irradiated mice as compared to control and non-irradiated aged mice. An
elevated IFN-γ/IL-4 ratio was also observed in aged senescence-accelerated
mouse-prone 1 (SAMP1) mice, a spontaneous skin photoaging model. Thus, an
imbalance between pro-inflammatory and anti-inflammatory cytokines might be
a key factor to differentiate photoaged skin from chronologically-aged skin.
Studying the pathogenesis of photoaged skin in humans is difficult because of
the decades needed for the evolution of this process, and the inability to assess
the total exposure to UV in a given individual [47]. Therefore, UV-irradiated
skh-hairless mice are widely used as an animal model for skin photoaging in the
elderly [15]. In this model, UV irradiation generally starts at 6 to 8 weeks of age,
and 10 to 22 weeks of irradiation are sufficient to produce human photoaged
skin-like lesions [16,17]. In other words, photoaged skin-like lesions are
developed at an adult age, but not in aged hairless mice; thus, an intrinsic
senescence process, which is essential for the manifestation of the phenotype of
photoaged skin in humans, seems to be unnecessary to reproduce the photoaged
skin-like lesions in hairless mice. These experimental conditions resulted in a
paucity of studies that have addressed chronological skin changes in hairless
Kligman et al., reported that skin from hairless mice at an advanced
(80-week-old) age without UV irradiation exhibited a sparse distribution of
elastic fibers in the dermis, and flocculent deposits of glycosaminoglycans
(GAGs) in the dermal-epidermal junctions (DEJ) [48]. These histological
changes were similar to chronologically-aged skin in photo-protected areas. Peres
et al., reported that there were quantitative and qualitative differences in
oxidative stress generated by chronological aging versus photoaging of hairless
mice [49]. The skin is intensively subjected to exogenous and endogenous
assaults; consequently, mechanisms concerning chronological aging and
photoaging remained to be elucidated.
In this chapter, I describe an attempt to investigate differences in the
histopathology and cytokine expression of non-irradiated skin at an advanced age
(70 weeks of age), and UV-irradiated and non-irradiated skin at a young age (18
weeks of age) in hairless mice to clarify factor(s) that differentiate photoaging
from chronological aging phenotypes in the skin.
Materials and Methods
Male albino hairless HOS: HR-1 hairless mice (6 weeks or 30 weeks of age)
were purchased from Hoshino Laboratory Animals Co., Ltd. (Saitama, Japan).
Mice were reared under conventional conditions, housed at 23 ± 2ºC, and were
allowed free access to food (CE2, Nihon CLEA, Tokyo, Japan) and tap water.
The light-dark cycle was set at 12 hours (lights were on at 07:00). Six-week-old
male hairless mice were acclimated for 2 weeks in the animal facility of Institute
for Developmental Research, Aichi Human Service Center, after which they
were subjected to UV irradiation. They were divided into UV-irradiated (n = 4)
and non-irradiated (control) (n = 4) groups. Thirty-week-old male hairless mice
were reared until 70 weeks of age without UV irradiation (aged mice) (n = 5). I
checked all of the mice pathologically after sampling of skin specimens, and
excluded samples from mice with inflammation-associated pathologies
(pneumonia and other inflammatory changes) and/or tumors from all subsequent
I took special care to minimize the number of animals used and their
suffering. All animals were handled in accordance with the Guide for the Care
and Use of Laboratory Animal of the Institute for Developmental Research,
Aichi Human Service Center.
UVB irradiation
UV irradiation was performed according to a method described by Schwartz
et al., [50] with some modifications. Briefly, a Handheld UV Lamp UVM-57
(UVP Inc., CA, USA) was used as a source of UVB. The wavelength of the
irradiated UV was 280-315 nm. A mouse was placed in a cage (12 × 9 × 3 cm)
and positioned at the center of the UVB source. The cage was covered by thin
wire netting such that the mice were evenly exposed to the UV. The UV
intensity was measured using a UV radiometer YK-34UV (Lutron Electronic
Co., Taipei, Taiwan) with spectral sensitivity within the range of 290-390 nm.
The dorsal skin of the mice was irradiated three times per week for 10 weeks.
The total irradiated dose was about 4 J/cm2. Skin tissues were removed two days
after the completion of the UV irradiation.
Histological examinations
Each mouse was sacrificed by cervical dislocation, and the dorsal skin was
rapidly removed, immersed in 10% neutral buffered formalin (pH 7.4) for 7 days,
and then embedded in paraffin. Twenty-micron-thick sections were cut with a
sliding microtome, and hematoxylin-eosin (H&E), resorcin-fuchsin and alcian
blue stainings were performed according to standard procedures.
The thickness of the epidermis was evaluated using sections stained with
H&E. Photomicrographs of continuous, non-overlapping visual fields (736 ×
533 μm) were obtained to cover the entire epidermis of the specimen using a
digital microscope (VHX-200, Keyence Corporation, Osaka, Japan). The
thickness of the epidermis was measured at 10 locations set at an interval of 70
μm apart, and the average thickness was calculated.
RNA extraction and real-time quantitative PCR
Dorsal skin was dissected from each mouse, and washed in ice-cold
phosphate-buffered saline (PBS). Total RNA was isolated using an ISOGEN kit
(NIPPON GENE CO., LTD., Tokyo, Japan) according to the manufacturer's
instructions. RNA yields and purities were determined by spectrophotometric
absorption analyses at 260/280 nm. The cDNA was synthesized from total RNA
using the SuperScriptTM III First-Strand Synthesis System for RT-PCR
(Invitrogen, Carlsbad, CA, USA) according to the manufacturer's instructions.
Gene expression was analyzed by a real time (RT) PCR system (ABI
Stepone-Plus, Applied Biosystems, Foster City, CA, USA) with each cDNA
sample, specific Taqman primers/probes and a Taqman Universal PCR Master
Mix (Applied Biosystems, Foster City, CA, USA). The following probes were
used (identified by Applied Biosystems assay identification number): IL-1 beta
(IL-1β), Mm00434228_m1; TNF-alpha (TNF-α), Mm00443258_m1; IL-6,
Mm00446190_m1; IFN-gamma (IFN- γ), Mm00801778_m1; TGF-beta1
(TGF-β1), Mm00441724_m1; iNOS, Mm00440485_m1; IL-4,
Mm00445260_m1; IL-10, Mm00439616_m1 and 18S rRNA, Hs99999901_s1.
18S rRNA was used as an internal control. The expression of these mRNA
species was analyzed using the same sets as for the total RNA samples. Reaction
mixtures were subjected to the following amplification scheme: 1 cycle at 50 ºC
for 2 min (AmpErase uracil-N-glycosylase deactivation) and 1 cycle at 95 ºC for
10 min (AmpliTaq Gold activation), followed by 40 cycles at 95 ºC for 15 s
(denaturation) and 60 ºC for 1 min (annealing and extension).
Statistical Analyses
Data were expressed as means ± S.D. A one-way analysis of variance
(ANOVA) followed by Tukey's procedure were performed to determine
differences in epidermal thickness and the expression of mRNA among control,
aged and UV-irradiated groups of hairless mice. Differences were considered to
be statistically significant when p < 0.05.
Histological analyses
In H&E-stained sections, spike-like downgrowths in the area of the DEJ
were evident in both control and aged mice (Fig. 9a, b). In contrast,
UV-irradiated mice showed an increase in the area of flattened DEJ (Fig.9c).
UV-irradiated mice, but not aged mice, showed marked thickening of the
epidermis (Fig. 9a, b, c). A one-way ANOVA showed a significant effect for
aging type (F(2,10) = 50.6086, p < 0.0001) in the epidermal thickness. Post-hoc
analyses revealed that the thickness of UV-irradiated mice epidermis (38.80 ±
5.85 μm) was significantly thicker than that in control mice (17.21 ±1.54 μm)
and that in aged mice (16.38 ± 2.42 μm). In contrast, there were no significant
differences between control and aged mice.
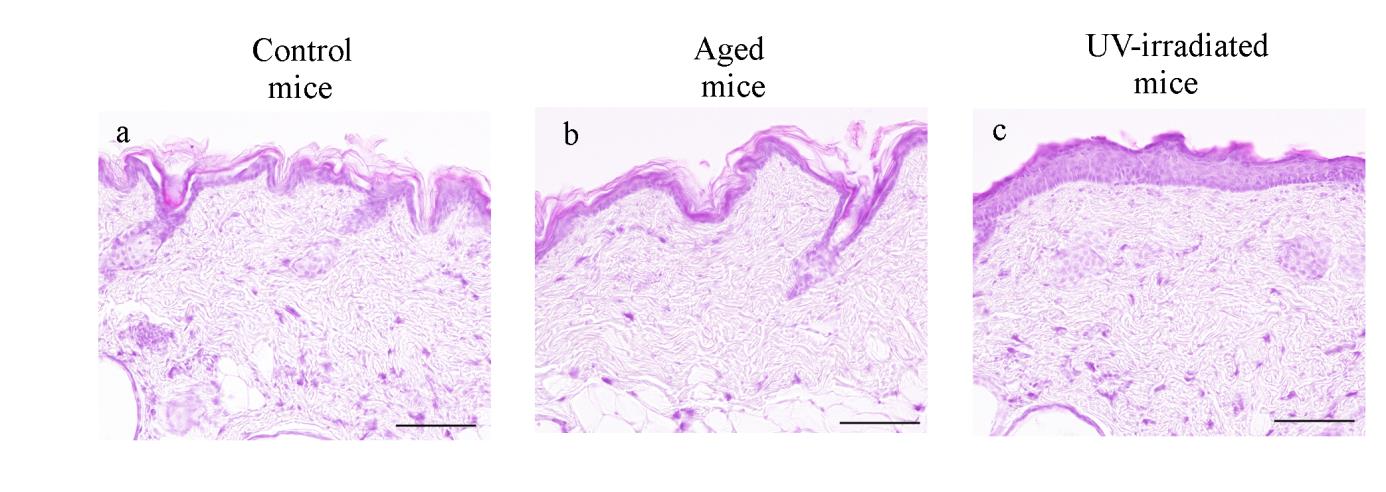
Fig. 9. Histological changes in the dorsal skin of hairless mice revealed by hematoxylin and
eosin (H&E) staining.
Representative photomicrographs of the dorsal skin from control (a), aged (b) and
UV-irradiated (c) hairless mice. Formalin-fixed skin sections were stained with H&E.
Photomicrographs were taken with a reduced condenser aperture. See the text for a detailed description. Scale bars: 100 μm.
In resorcin-fuchsin-stained sections, fine elastic fibers just beneath the
epidermis were fewer in aged mice (Fig.10a, b, open arrowheads). The elastic
fibers in the reticular dermis were thickened in aged mice as compared to
control mice (Fig. 10b, thin arrow). In the UV-irradiated mice, the above
mentioned changes were more pronounced as compared to control mice (Fig.
10c, open arrowhead and thin arrow).
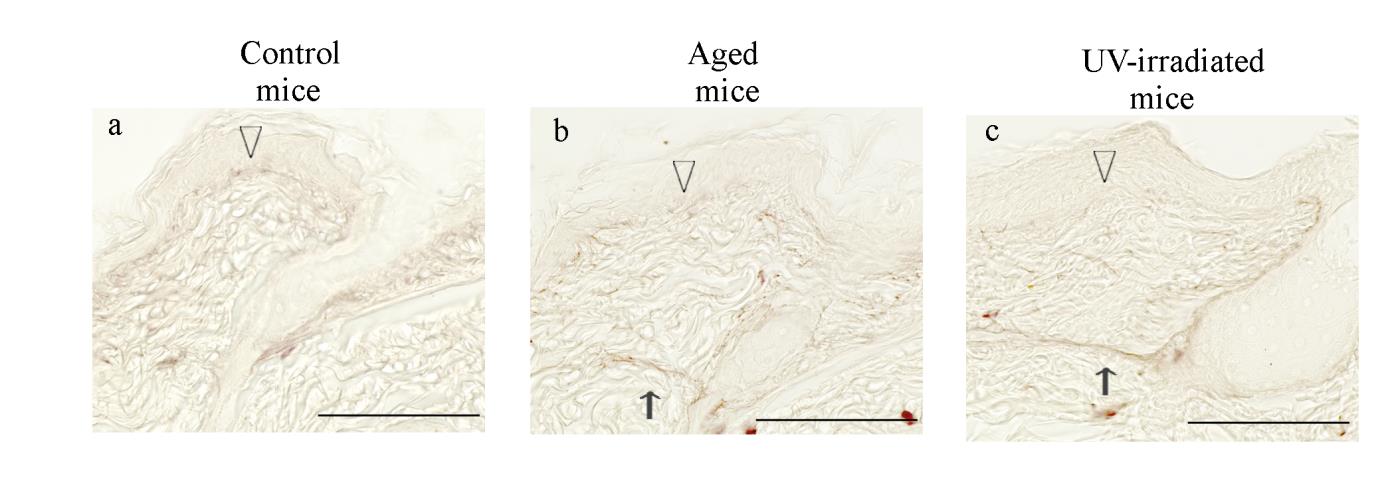
Fig. 10. Histological changes in the dorsal skin of hairless mice revealed by resorcin-fuchsin
Representative photomicrographs of the dorsal skin from control (a), aged (b) and
UV-irradiated (c) hairless mice. Formalin-fixed skin sections were stained with
resorcin-fuchsin. The open arrowheads indicate fine elastic fibers in the dermal-epidermal
junction, and the thin arrows indicate thickened elastic fibers in the reticular dermis.
Photomicrographs were taken with a reduced condenser aperture. See the text for a detailed
description. Scale bars: 100 μm.
In alcian blue-stained sections, a slight accumulation of alcian blue-positive
GAGs was observed just beneath the epidermis of aged mice (Fig. 11b, closed
arrowheads). The UV-irradiated mice showed a more prominent accumulation
of GAGs just beneath the epidermis (Fig. 11c, closed arrowheads).
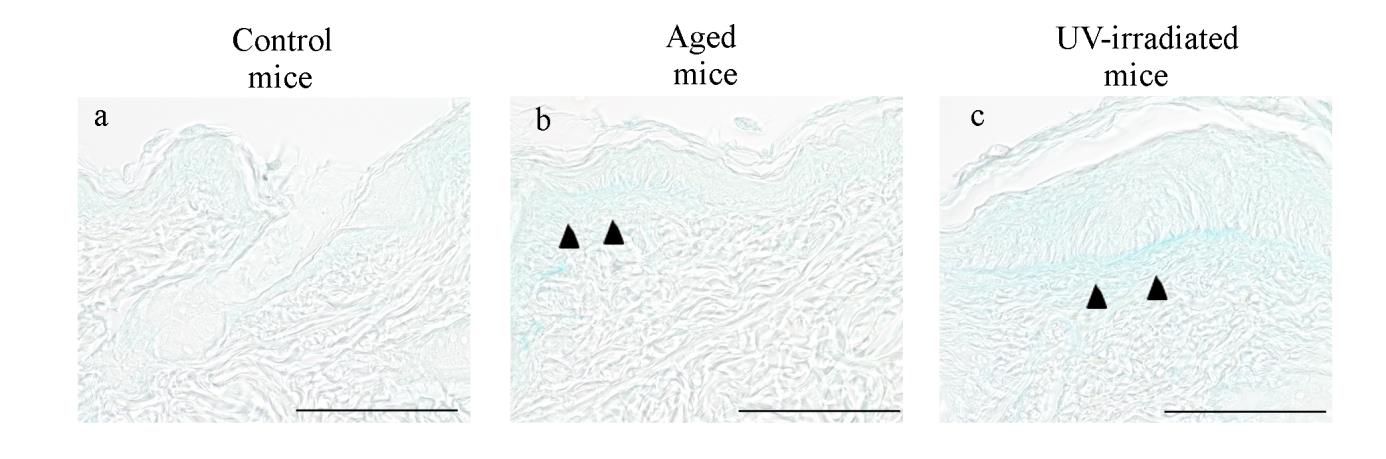
Fig. 11. Histological changes in the dorsal skin of hairless mice revealed by alcian blue
Representative photomicrographs of the dorsal skin from control (a), aged (b) and
UV-irradiated (c) hairless mice. Formalin-fixed skin sections were stained with alcian blue.
The closed arrowheads in (b) and (c) indicate increased glycosaminoglycans.
Photomicrographs were taken with a reduced condenser aperture. See the text for a detailed description. Scale bars: 100 μm.
Gene expression analyses of hairless mouse skin by real-time PCR
A one-way ANOVA revealed no significant effect for aging type (control,
UV-irradiated and chronologically-aged) in the expression of IL-1β mRNA
(F(2,10) = 1.0554, p = 0.3838) (Fig. 12a).
In the expression of TNF-α mRNA, a one-way ANOVA showed a
significant effect for the aging type (F(2,10) = 8.3231, p = 0.0074). TNF-α
mRNA expression in aged mice was significantly greater than in control and
UV-irradiated mice, whereas there was no significant difference between control
and UV-irradiated mice (Fig. 12b).
Fig. 12. Changes in the expression of IL-1β and TNF-α mRNA in the skin from hairless mice.
The expression of IL-1β (a) and TNF-α (b) mRNAs in the skin from control, aged and
UV-irradiated hairless mice were analyzed by real-time PCR. The relative levels of mRNA
were obtained by dividing the quantity of each mRNA by that of 18S rRNA as the internal
standard. All data are presented as means ± S.D. The number of mice used in this experiment
ranged from 4 to 5 for each experimental group. (*) p < 0.05.
A one-way ANOVA revealed no significant effect for aging type (control,
UV-irradiated and chronologically-aged) in the expression of IL-6 mRNA
(F(2,10) = 2.8796, p = 0.1029) (Fig. 13a).
In the expression of IFN-γ mRNA, a one-way ANOVA showed a significant
effect for the aging type (F(2,10) = 12.0917, p = 0.0021). Tukey's procedure
revealed significantly greater IFN-γ mRNA expression in UV-irradiated mice as
compared to control (5.8-fold) and aged (2.0-fold) mice (Fig. 13b).
Fig. 13. Changes in the expression of IL-6 and IFN-γ mRNA in the skin from hairless mice.
The expression of IL-6 (a) and IFN-γ (b) mRNAs in the skin from control, aged and
UV-irradiated hairless mice were analyzed by real-time PCR. The relative levels of mRNA
were obtained by dividing the quantity of each mRNA by that of 18S rRNA as the internal
standard. All data are presented as means ± S.D. The number of mice used in this experiment
ranged from 4 to 5 for each experimental group. (*) p < 0.05, (**) p < 0.01.
In the expression of TGF-β1 mRNA, a one-way ANOVA showed a
significant effect for the aging type (F(2,10) = 29.3000, p = 0.0001). TGF-β
mRNA levels in aged mice were significantly greater than those in control and
UV-irradiated mice. No significant differences were detected between control
and UV-irradiated mice (Fig. 14a).
In the expression of iNOS mRNA, a one-way ANOVA showed a significant
effect for the aging type (F(2,10) = 23.4744, p = 0.0002). iNOS mRNA levels in
aged mice were significantly greater than those in control and UV-irradiated
mice. No significant differences were detected between control and
UV-irradiated mice (Fig. 14b).
Fig. 14. Changes in the expression of TGF-β1 and iNOS mRNA in the skin from hairless
The expression of TGF-β1 (a) and iNOS (b) mRNAs in the skin from control, aged and
UV-irradiated hairless mice were analyzed by real-time PCR. The relative levels of mRNA
were obtained by dividing the quantity of each mRNA by that of 18S rRNA as the internal
standard. All data are presented as means ± S.D. The number of mice used in this experiment
ranged from 4 to 5 for each experimental group. (**) p < 0.01.
In the expression of IL-4 mRNA, a one-way ANOVA showed a significant
effect for the aging type (F(2,10) = 5.0001, p = 0.0312). IL-4 mRNA levels in
aged mice were 3.3-fold higher than those in control mice. In contrast, no
significant differences were detected between control and UV-irradiated mice
In the expression of IL-10, a one-way ANOVA showed a significant effect
for the aging type (F(2,10) = 6.7659, p = 0.0139). IL-10 mRNA levels in aged
mice were 3.4-fold greater than those in control mice. In contrast, no significant
differences were detected between control and UV-irradiated mice (Fig. 15b).
Fig. 15. Changes in the expression of IL-4 and IL-10 mRNA in the skin from hairless mice.
The expression of IL-4 (a) and IL-10 (b) mRNAs in the skin from control, aged and
UV-irradiated hairless mice were analyzed by real-time PCR. The relative levels of mRNA
were obtained by dividing the quantity of each mRNA by that of 18S rRNA as the internal
standard. All data are presented as means ± S.D. The number of mice used in this experiment
ranged from 4 to 5 for each experimental group. (*) p < 0.05.
To evaluate the balance between pro-inflammatory and
anti-inflammatory cytokines, I compared the IFN-γ/IL-4 ratio among
control, aged and UV-irradiated mice (Fig. 16). A one-way ANOVA
showed a significant effect for the aging type (F(2,10) = 40.6563, p <
0.0001). The IFN-γ/IL-4 ratio was significantly greater in UV-irradiated
mice than in control and aged mice. In contrast, there was no significant
difference in the IFN-γ/IL-4 ratio between control and aged mice (Fig. 16).
Fig. 16. Changes in the IFN-γ/IL-4 ratio in the skin from hairless mice.
The IFN-γ/IL-4 ratio from control, aged and UV-irradiated hairless mice were indicated.
All data are presented as means ± S.D. The number of mice used in this experiment
ranged from 4 to 5 for each experimental group. (**) p < 0.01.
To evaluate whether the imbalance between pro-inflammatory and
anti-inflammatory cytokines may contribute to the pathogenesis of
photoaged skin phenotype, I compared the IL-4 mRNA expression and
IFN-γ/IL-4 ratio in the skin from SAMP1 mice, a spontaneous photoaging
model, and control SAMR1 mice.
In the expression of IL-4 mRNA, a one-way ANOVA showed a
significant effect for age in SAMP1 mice (F(5,28) = 9.6324, p < 0.0001).
The expression of IL-4 mRNA increased dramatically towards 48 weeks of
age, but then decreased at 70 weeks of age. The expression level of IL-4
mRNA at 48 weeks of age was about 10-fold higher than that at 12 weeks
of age. A two-way ANOVA showed a significant interaction between the
effects of strain and age on the expression of IL-4 (F(1,14) = 13.1949, p =
0.0027). Simple main effects analysis revealed that 70-week-old SAMR1
mice showed greater expression than that in SAMP1 mice at the same age,
whereas there were no differences between 12-week-old SAMP1 and
SAMR1 mice (Fig. 17a).
In the ratio of IFN-γ/IL-4, a one-way ANOVA showed a significant
effect for age in SAMP1 mice (F(5,28) = 41.8817, p < 0.0001). The
IFN-γ/IL-4 ratio at 70 weeks of age was 107.5-fold higher than that at 12
weeks of age. A two-way ANOVA showed a significant interaction between
the effects of strain and age on the IFN-γ/IL-4 ratio (F(1,14) = 28.4944, p =
0.0001). Simple main effects analysis revealed that 70-week-old SAMP1
mice showed greater IFN-γ/IL-4 ratio than that in SAMR1 mice at the same
age, whereas there were no differences between 12-week-old SAMP1 and
SAMR1 mice (Fig. 17b).
Fig. 17. Expression changes in IL-4 mRNA and IFN-γ/IL-4 ratio in the skin from
SAMP1 and SAMR1 mice.
The expression of IL-4 mRNA (a) in the skin from SAMP1 and SAMR1 mice were
analyzed by real-time PCR. The relative levels of mRNA were obtained by dividing the
quantity of each mRNA by that of 18S rRNA as the internal standard. (b) indicates
IFN-γ/IL-4 ratio. The closed bars represent SAMP1 mice, and the open bars represent
SAMR1 mice. All data are presented as means ± S.D. The number of mice used in this
experiment ranged from 4 to 11 for each experimental group. For the detailed materials
and methods, see chapter 1. (*) p < 0.05, (**) p < 0.01.
Discussion
In the present study, I showed that aged (70-week-old) hairless mice
exhibited minimal changes in their dermal elastic fibers and GAGs without
epidermal thickening. These changes were similar to those in a previous
report by Kligman et al., [48] and to the pathology of chronologically-aged
skin in photo-protected areas in humans, except for the absence of
flattening of DEJ [11]. In contrast, I were able to reproduce photoaging-like
histological changes, such as alterations in dermal elastic fibers, the
deposition of GAG and thickening of the epidermis [2,3], in hairless mice
by UV irradiation for 10 weeks. Thus, chronological factors may have little,
if any, contribution to the pathogenesis of the photoaging-like phenotype in
hairless mice, and UV irradiation seems to be a necessary and sufficient
ROS, pro-inflammatory cytokines and MMPs are thought to be key
factors for the pathogenesis of photoaging [1,9]. On the other hand, these
factors also contribute to the intrinsic aging process of the skin [51-53].
Accumulating evidence suggests that photoaging and chronological aging
share some important molecular features, and photoaging can be regarded
as the superposition of solar damage on the normal aging process [2,53]. To
investigate differentiating molecular factor(s) between photoaging and
chronological aging, I focused on the pattern of cytokine expression in
hairless mice models for these two aging phenotypes. I found that
chronologically aged hairless mice showed an increase in the expression of
both pro-inflammatory molecules, such as TNF-α and iNOS, and
anti-inflammatory molecules, such IL-4 and IL-10. In contrast,
UV-irradiated hairless mice showed a marked increase in IFN-γ expression,
but no significant increase in anti-inflammatory cytokines. A balanced
production of pro-inflammatory and anti-inflammatory cytokines is
important for an appropriate immune response [54-56], and an imbalance
between pro-inflammatory and anti-inflammatory cytokines has been
reported in psoriasis, a chronic inflammatory skin disease caused by
autoimmune mechanisms [57]. To evaluate the balance between
pro-inflammatory and anti-inflammatory cytokines in these two aging
phenotypes, I calculated the IFN-γ/IL-4 ratio [54,58]. In
chronologically-aged skin, the IFN-γ/IL-4 ratio was similar to that in
control mice, suggesting that the pro-inflammatory/anti-inflammatory
balance is maintained in aged hairless mice. In contrast, UV-irradiated
hairless mice showed a significant increase in their IFN-γ/IL-4 ratio. Thus,
although not all pro-inflammatory cytokines were increased in
UV-irradiated mice, the imbalance between pro-inflammatory versus
anti-inflammatory cytokines might be a differentiating factor between the
two aging phenotypes in the skin.
To confirm the association between the skin photoaging phenotype and
the shift to pro-inflammatory status, I evaluated the IFN-γ/IL-4 ratio in
SAMP1 mice (Fig. 17b). The IFN-γ/IL-4 ratio of the skin from
70-week-old SAMP1 mice was markedly increased as compared to that of
12-week-old SAMP1 mouse skin. In contrast, no significant difference was
observed between 12- and 70-weeks of age the IFN-γ/IL-4 ratios in
SAMR1 mice. Thus, the imbalance between pro-inflammatory and
anti-inflammatory cytokines seems to be a common feature of
UV-irradiated hairless mice and old SAMP1 mice, which are both skin
photoaging models.
Conclusion
Based on the results of this study, a SAMP1 strain of mice with a
higher oxidative status is proposed as a new animal model for human skin
photoaging due to exaggerated intrinsic factors. The skin from old SAMP1
mice exhibited phenotypes closely resembling human photoaged skin, in
terms of histological changes, the expression changes of cytokines and
MMPs. Several antioxidants are available in topical and oral preparations
to prevent or treat photoaging [46]. Since SAMP1 mice are a unique model
which develops photoaging-like phenotypes due to higher oxidative stress,
the effects of antioxidants may be observed more clearly than in an
UV-irradiation model. The SAMP1 mouse strain can be a useful
spontaneous animal model for investigating the pathogenic mechanisms of
human photoaging, especially the mechanistic link among the intrinsic
aging process, oxidative stress and photoaging phenotypes.
An attempt was made to clarify factor(s) that differentiate photoaging
from chronological aging phenotypes. Histological changes were compared
with cytokine expression patterns among UV-irradiated hairless mice (18
weeks of age), a standard photoaging model, non-irradiated mice of 18
weeks of age and chronologically-aged hairless mice (70 weeks of age).
Chronologically-aged skin and photoaged skin in hairless mice exhibited
different histological and gene expression changes, and an imbalance
between pro-inflammatory and anti-inflammatory cytokines, indicated by
an elevated IFN-γ/IL-4 ratio, which might be a key factor to differentiate
these two phenotypes of skin aging. Interestingly, SAMP1 mice also
showed increased IFN-γ/IL-4 ratio with advancing age. Finally, an
imbalance between pro-inflammatory and anti-inflammatory conditions
caused by UV and/or ROS is proposed as a mechanism of skin photoaging.
How the pro-inflammatory/anti- inflammatory imbalance affects
phenotypes of photoaged skin deserves further investigation. These novel
results contribute towards the fundamental understanding of the regulatory
mechanisms of photoaged skin and chronologically-aged skin in
photo-protected skin. These results can help determine if photoaging is a
pathological process or part of the normal aging process.
I thank Professor Minoru Takeuchi for his guidance and
encouragement throughout this work. I wish to thank Dr. Masanori
Hosokawa, Dr. Yoichi Chiba, Dr. Yasushi Enokido and Dr. Ayako
Furukawa for their suggestions and excellent technical helps in this study. I
also thank Ms. Noriko Kawamura, Ms. Tomoko Kitajima, Ms. Takae
Hiraide and Ms. Emi Kamiya for their technical assistance. In the last, I
would like to express my sincere thanks to the members in our company for
their encouragements and valuable suggestions.
References
[1] Rabe J H, Mamelak A J, McElgunn P J S, Morison W L, Sauder D N. Photoaging:
mechanisms and repair. J Am Acad Dermatol 2006: 55: 1-19.
[2] Farage M A, Miller K W, Elsner P, Maibach H I. Intrinsic and extrinsic factors in
skin ageing: a review. Int J Cosmet Sci 2008: 30: 87-95.
[3] Kligman L H, Kligman A M. The nature of photoaging: its prevention and repair.
Photodermatol 1986: 3: 215-227.
[4] Berneburg M, Plettenberg H, Krutman J. Photoaging of human skin. Photodermatol
Photoimmunol Photomed 2000: 16: 239-244.
[5] Epstein J H. Photocarcinogenesis, skin cancer, and aging. In: Balin A K, Kligman
A M, eds. Aging and the skin. New York: Raven Press, 1989: 307-329.
[6] Sugimoto M, Yamashita R, Ueda M. Telomere length of the skin in association
with chronological aging and photoaging. J Dermatol Sci 2006: 43: 43-47.
[7] Svobodová A, Psotová J, Walterová D. Natural phenolics in the prevention of
UV-induced skin damage. A review. Biomed Papers 2003: 147: 137-145.
[8] Kulka M. Mechanisms and treatment of photoaging and photodamage. In: Kulka M,
eds. Using old solutions to new problem – natural drug discovery in the 21st
century. InTech, 2013: 255-276.
[9] Yaar M, Gilchrest B A. Photoageing: mechanism, prevention and therapy. Br J
Dermatol 2007: 157: 874-887.
[10] Rijken F, Bruijnzeel P L. The pathogenesis of photoaging: the role of neutrophils
and neutrophil-derived enzymes. J Investig Dermatol Symp Proc 2009: 14: 67-72.
[11] Mukherjee S, Date A, Patravale V, Korting H C, Roeder A, Weindl G. Retinoids in
the treatment of skin aging: an overview of clinical efficacy and safety. Clin Interv
Aging 2006: 1: 327-348.
[12] Werth B B, Bashir M, Chang L, Werth V P. Ultraviolet irradiation induces the
accumulation of chondroitin sulfate, but not other glycosaminoglycans, in human
skin. PLoS One 2011: 6: e14830. doi:10.1371/journal.pone.0014830.
[13] Lee J Y, Kim Y K, Seo J Y et al. Loss of elastic fibers causes skin wrinkles in
sun-damaged human skin. J Dermatol Sci 2008: 50: 99-107.
[14] Yaar M. Clinical and histological features of intrinsic versus extrinsic skin aging.
In: Gilchrest B A, Krutmann J, eds. Skin Aging. Heidelberg: Springer-Verlag,
[15] Benavides F, Oberyszyn T M, VanBuskirk A M, Reeve V E, Kusewitt D F. The
hairless mouse in skin research. J Dermatol Sci 2009: 53: 10-18.
[16] Inomata S, Matsunaga Y, Amano S et al. Possible involvement of gelatinases in
basement membrane damage and wrinkle formation in chronically ultraviolet
B-exposed hairless mouse. J Invest Dermatol 2003: 120: 128-134.
[17] Ropke C D, Sawada T C, da Silva V V, Michalany N S, de Moraes Barros S B.
Photoprotective effect of Pothomorpheumbellata root extract against ultraviolet
radiation induced chronic skin damage in the hairless mouse. Clin Exp Dermatol
2005: 30: 272-276.
[18] Chiba Y, Fujisawa H, Nishikawa T, Hosokawa M. Higher oxidative stress status
and mitochondrial alterations as a possible mechanism for senescence acceleration.
In: Takeda T, Akiguchi I, Higuchi K, Hosokawa M, Hosokawa T, Nomura Y, eds.
The senescence-accelerated mouse (SAM): achievements and future directions.
Amsterdam: Elsevier, 2013: 373-387.
[19] Chiba Y, Shimada A, Kumagai N et al. The senescence-accelerated mouse (SAM):
a higher oxidative stress and age-dependent degenerative diseases model.
Neurochem Res 2009: 34: 679-687.
[20] Nomura Y, Takeda T, Okuma Y. 2004. The senescence-accelerated mouse (SAM):
An animal model of senescence. Amsterdam: Elsevier.
[21] Takeda T, Hosokawa M, Takeshita S et al. A new murine model of accelerated
senescence. Mech Ageing Dev 1981: 17: 183-194.
[22] Takeda T. Senescence-accelerated mouse (SAM): a biogerontological resource in
aging research. Neurobiol Aging 1999: 20: 105-110.
[23] Chiba Y, Yamashita Y, Ueno M et al. Cultured murine dermal fibroblast-like cells
from senescence-accelerated mice as in vitro models for higher oxidative stress due
to mitochondrial alterations. J Gerontol A Biol Sci Med Sci 2005: 60: 1087-1098.
[24] Hosokawa M. A higher oxidative status accelerates senescence and aggravates
age-dependent disorders in SAMP strains of mice. Mech Ageing Dev 2002: 123:
[25] Hosokawa M, Ashida Y, Nishikawa T, Takeda T. Accelerated aging of dermal
fibroblast-like cells from senescence-accelerated mouse (SAM). 1. Acceleration of
population aging in vitro. Mech Ageing Dev 1994: 74: 65-77.
[26] Fujisawa H, Nishikawa T, Zhu B H et al. Aminoguanidine supplementation delays
the onset of senescence in vitro in dermal fibroblast-like cells from
senescence-accelerated mice. J Gerontol A Biol Sci Med Sci 1995: 54: 276-282.
[27] Komura S, Yoshino K, Kondo K, Yagi K. Lipid peroxide levels in the skin of the
senescence-accelerated mouse. J Clin Biochem Nutr 1988: 5: 255-260.
[28] Okada T, Hayakawa R, Yoshino K et al. Deposition of lipofuscin and elastic fibers
in the skin of the senescence-accelerated mouse. J Clin Biochem Nutr 1990: 9:
[29] Kumagai N, Chiba Y, Hosono M et al. Involvement of pro-inflammatory cytokines
and microglia in an age-associated neurodegeneration model, the SAMP10 mouse.
Brain Res 2007: 1185: 75-85.
[30] Ohkawa H, Ohishi N, Yagi K. Assay for lipid peroxides in animal tissues by
thiobarbituric acid reaction. Anal Biochem 1979: 95: 351-358.
[31] Pillai S, Oresajo C, Hayward J. Ultraviolet radiation and skin aging: roles of
reactive oxygen species, inflammation and protease activation, and strategies for
prevention of inflammation-induced matrix degradation- a review. Int J Cosmet Sci
2005: 27: 17-34.
[32] Takashima A, Bergstresser P R. Impact of UVB radiation on the epidermal
cytokine network. Photochem Photobiol 1996: 63: 397-400.
[33] Kupper T S, Chua A O, Flood P, McGuire J, Gubler U. Interleukin 1 gene
expression in cultured human keratinocytes is augmented by ultraviolet irradiation.
J Clin Invest 1987: 80: 430-436.
[34] Köck A, Schwarz T, Kirnbauer R et al. Human keratinocytes are a source for tumor
necrosis factor α: evidence for synthesis and release upon stimulation with
endotoxin or ultraviolet light. J Exp Med 1990: 172: 1609-1614.
[35] de Vos S, Brach M, Budnik A, Grewe M, Herrmann F, Krutmann J.
Post-transcriptional regulation of interleukin-6 gene expression in human
keratinocytes by ultraviolet B radiation. J Invest Dermatol 1994: 103: 92-96.
[36] Urbanski A, Schwarz T, Neuner P et al. Ultraviolet light induces increased
circulating interleukin-6 in humans. J Invest Dermatol 1990: 94: 808-811.
[37] Terui T, Tagami H. Mediators of inflammation involved in UVB erythema. J
Dermatol Sci 2000: 23 (Suppl 1): S1-5.
[38] Tha K K, Okuma Y, Miyazaki H et al. Changes in expressions of pro-inflammatory
cytokines IL-1β, TNF-α and IL-6 in the brain of senescence accelerated mouse
(SAM) P8. Brain Res 2000: 885: 25-31.
[39] Wang H, Kochevar I E. Involvement of UVB-induced reactive oxygen species in
TGF-β biosynthesis and activation in keratinocytes. Free Radic Biol Med 2005: 38:
[40] Quan T, He T, Kang S, Voorhees J J, Fisher G J. Ultraviolet irradiation alters
transforming growth factor β/smad pathway in human skin in vivo. J Invest
Dermatol 2002: 119: 499-506.
[41] Suschek C V, Bruch-Gerharz D, Kleinert H, Förstermann U, Kolb-Bachofen V.
Ultraviolet A1 radiation induces nitric oxide synthase-2 expression in human skin
endothelial cells in the absence of pro-inflammatory cytokines. J Invest Dermatol
2001: 117: 1200-1205.
[42] Nishigori C, Hattori Y, Arima Y, Miyachi Y. Photoaging and oxidative stress. Exp
Dermatol 2003: 12 (Suppl 2): 18-21.
[43] Saarialho-Kere U, Kerkelä E, Jeskanen L et al. Accumulation of matrilysin
(MMP-7) and macrophage metalloelastase (MMP-12) in actinic damage. J Invest
Dermatol 1999: 113: 664-672.
[44] Vaalamo M, Kariniemi A L, Shapiro S D, Saarialho-Kere U. Enhanced expression
of human metalloelastase (MMP-12) in cutaneous granulomas and macrophage
migration. J Invest Dermatol 1999: 112: 499-505.
[45] Krutmann J, Schroeder P. Role of mitochondria in photoaging of human skin: the
defective powerhouse model. J Investig Dermatol Symp Proc 2009: 14: 44-49.
[46] Lin F H, Lin J Y, Gupta R D et al. Ferulic acid stabilizes a solution of vitamins C
and E and doubles its photoprotection of skin. J Invest Dermatol 2005: 125:
[47] Kligman, L H. The hairless mouse model for photoaging. Clin Dermatol 1996: 14:
[48] Kligman L H, Mezick J A, Capetola R J, Thorne E G. Lifetime topical application
of tretinoin to hairless mice. Acta Derm Venereol 1992: 72: 418-422.
[49] Peres P S, Terra V A, Guarnier F A, Cecchini R, Cecchini A L. Photoaging and
chronological aging profile: Understanding oxidation of the skin. Journal
Photochem Photobiol B Biol 2011: 103: 93-97.
[50] Schwartz E, Sapadin A N, Kligman L H. Ultraviolet B radiation increases
steady-state mRNA levels for cytokines and integrins in hairless mouse skin:
modulation by topical tretinoin. Arch Dermatol Res 1998: 290: 137-144.
[51] Quan T, Qin Z, Robichaud P,Voorhees J J, Fisher G J. CCN1 contributes to skin
connective tissue aging by inducing age-associated secretory phenotype in human
skin dermal fibroblasts. J Cell Commun Signaling 2011: 5: 201-207.
[52] Varani J, Warner R L, Gharaee-Kermani M et al. Vitamin A antagonizes decreased
cell growth and elevated collagen-degrading matrix metalloproteinases and
stimulates collagen accumulation in naturally aged human skin. J Invest Dermatol
2000: 114: 480-486.
[53] Fisher G J, Kang S, Varani J et al. Mechanisms of photoaging and chronological
skin aging. Arch Dermatol 2002: 138: 1462-1470.
[54] Hernandez Cruz A , Garcia-Jimenez S, Zucatelli Mendonca R, Petricevich V L.
Pro- and anti-inflammatory cytokines release in mice injected with Crotalus
durissus terrificus venom. Mediators Inflamm 2008; 874962.
[55] Nyati K K, Prasad K N, Rizwan A, Verma A, Paliwal V K. TH1 and TH2 response
to Campylobacter jejuni antigen in Guillain-Barre syndrome. Arch Neurol 2011:
[56] Kidd P. Th1/Th2 balance: the hypothesis, its limitations, and implications for
health and disease. Altern Med Rev 2003: 8: 223-246.
[57] Asadullah K, Sterry W, Stephanek K et al. IL-10 is a key cytokine in psoriasis.
Proof of principle by IL-10 therapy: a new therapeutic approach. J Clin Invest
1998: 101: 783-794.
[58] Kim Y K, Jung H G, Myint A M, Kim H, Park S H. Imbalance between
pro-inflammatory and anti-inflammatory cytokines in bipolar disorder. J Affect
Disord 2007: 104: 91-95.
Source: https://ksurep.kyoto-su.ac.jp/dspace/bitstream/10965/1246/2/DT_B_38_5_2.pdf
Serum TNF-α, IL-10 and IL-2 in Schizophrenic Patients Before and After Treatment with Risperidone and Clozapine Abolghasem Ajami1, Farshideh Abedian2*, Seyyed Hamzeh Hosseini3, Elahe Akbarian4, Reza Alizadeh-Navaei1, Mehrdad Taghipour5 1Molecular and Cell Biology Research Center, 2Department of Immunology, 3Psychiatry Research Center,
Reproductive Technologies: Royal Commission Final Report(MR-124e) ROYAL COMMISSION FINAL REPORT Prepared by Nancy Miller Chenier Political and Social Affairs Division 22 April 1994 TABLE OF CONTENTS PART ONE: REPRODUCTIVE TECHNOLOGIES AND CANADIAN SOCIETY PART TWO: CONDITIONS, TECHNOLOGIES, AND PRACTICES A. Prevalence, Risk Factors and Prevention of Infertility